CHAPTER II DESCRIPTIONS OF NUCLEAR EXPLOSIONS
INTRODUCTION
2.01 A number of characteristic phenomena, some of which are visible whereas others are not directly apparent, are associated with nuclear explosions. Certain aspects of these phenomena will depend on the type of burst, i.e., air, high-altitude, surface, or subsurface, as indicated in Chapter I. This dependence arises from direct and secondary interactions of the output of the exploding weapon with its environment, and leads to variations in the distribution of the energy released, particularly among blast, shock, and thermal radiation. In addition, the design of the weapon can also affect the energy distribution. Finally, meteorological conditions, such as temperature, humidity, wind, precipitation, and atmospheric pressure, and even the nature of the terrain over which the explosion occurs, may influence some of the observed effects. Nevertheless, the gross phenomena associated with a particular type of nuclear explosion, namely, high-altitude, air, surface, underwater, or underground, remain unchanged. It is such phenomena that are described in this chapter.
2.02 The descriptions of explosions at very high altitudes as well as those in the air nearer to the ground refer mainly to nuclear devices with energies in the vicinity of 1-megaton TNT equivalent. For underwater bursts, the information is based on the detonations of a few weapons with roughly 20 to 30 kilotons of TNT energy in shallow and moderately deep, and deep water. Indications will be given of the results to be expected for explosions of other yields. As a general rule, however, the basic phenomena for a burst in a particular environment are not greatly dependent upon the energy of the explosion. In the following discussion it will be supposed, first, that a typical air burst takes place at such a height that the fireball, even at its maximum, is well above the surface of the earth. The modifications, as well as the special effects, resulting from a surface burst and for one at very high altitude will be included. In addition, some of the characteristic phenomena associated with underwater and underground nuclear explosions will be described.
DESCRIPTION OF AIR AND SURFACE BURSTS
THE FIREBALL
2.03 As already seen, the fission of uranium (or plutonium) or the fusion of the isotopes of hydrogen in a nuclear weapon leads to the liberation of a large amount of energy in a very small period of time within a limited quantity of matter. As a result, the fission products, bomb casing, and other weapon parts are raised to extremely high temperatures, similar to those in the center of the sun. The maximum temperature attained by the fission weapon residues is several tens of million degrees, which may be compared with a maximum of 5,000°C (or 9,000°F) in a conventional high-explosive weapon. Because of the great heat produced by the nuclear explosion, all the materials are converted into the gaseous form. Since the gases, at the instant of explosion, are restricted to the region occupied by the original constituents in the weapon, tremendous pressures will be produced. These pressures are probably over a million times the atmospheric pressure, i.e., of the order of many millions of pounds per square inch.
2.04 Within less than a millionth of a second of the detonation of the weapon, the extremely hot weapon residues radiate large amounts of energy, mainly as invisible X rays, which are absorbed within a few feet in the surrounding (sea-level) atmosphere (§ 1.78). This leads to the formation of an extremely hot and highly luminous (incandescent) spherical mass of air and gaseous weapon residues which is the fireball referred to in § 1.32; a typical fireball accompanying an air burst is shown in Fig. 2.04. The surface brightness decreases with time, but after about a millisecond,1 the fireball from a 1-megaton nuclear weapon would appear to an observer 50 miles away to be many times more brilliant than the sun at noon. In several of the nuclear tests made in the atmosphere at low altitudes at the Nevada Test Site, in all of which the energy yields were less than 100 kilotons, the glare in the sky, in the early hours of the dawn, was visible 400 (or more) miles away. This was not the result of direct (line-of-sight) transmission, but rather of scattering and diffraction, i.e., bending, of the light rays by particles of dust and possibly by moisture in the atmosphere. However, high-altitude bursts in the megaton range have been seen directly as far as 700 miles away.
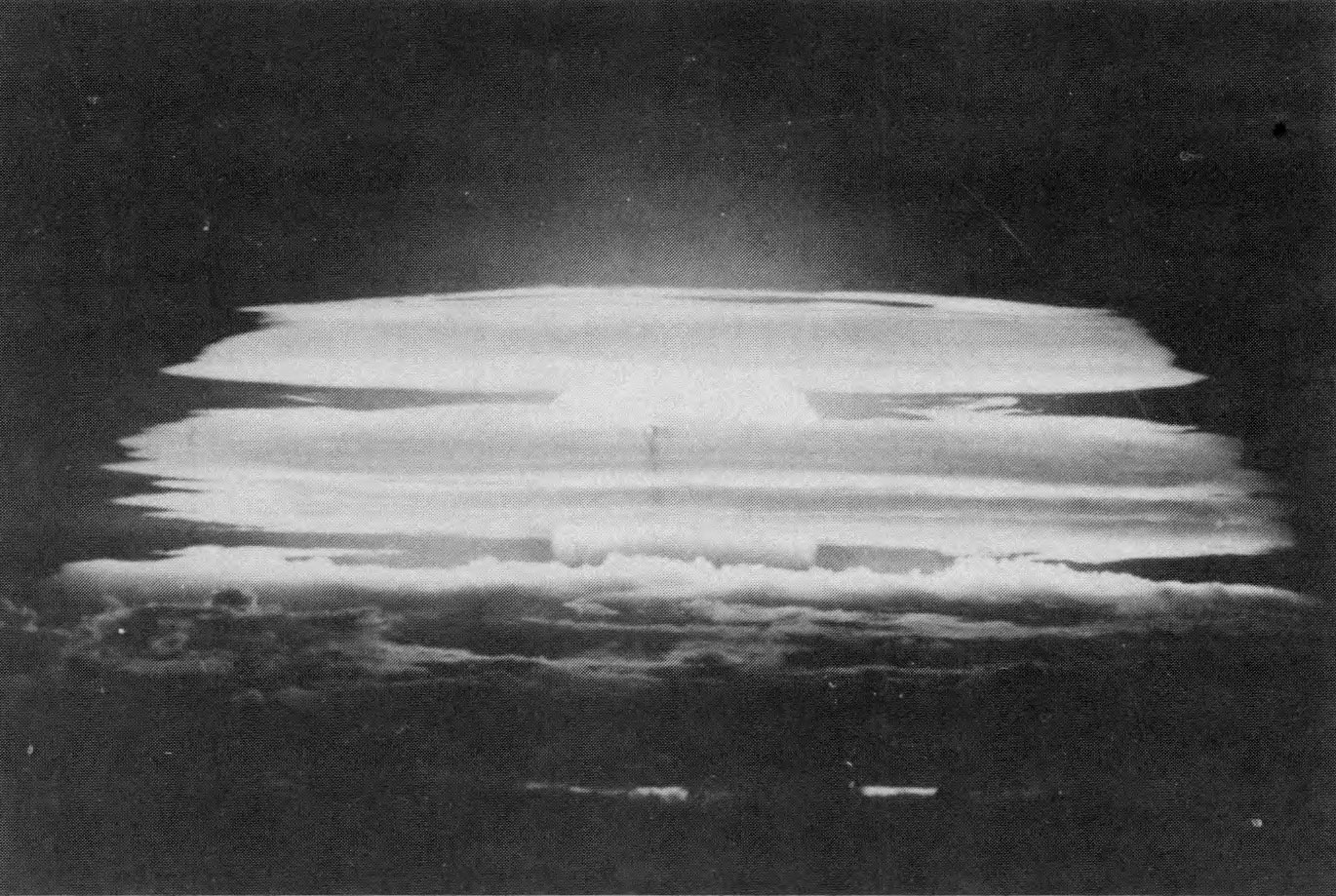
2.05 The surface temperatures of the fireball, upon which the brightness (or luminance) depends, do not vary greatly with the total energy yield of the weapon. Consequently, the observed brightness of the fireball in an air burst is roughly the same, regardless of the amount of energy released in the explosion. Immediately after its formation, the fireball begins to grow in size, engulfing the surrounding air. This growth is accompanied by a decrease in temperature because of the accompanying increase in mass. At the same time, the fireball rises, like a hot-air balloon. Within seven-tenths of a millisecond from the detonation, the fireball from a 1-megaton weapon is about 440 feet across, and this increases to a maximum value of about 5, 700 feet in 10 seconds. It is then rising at a rate of 250 to 350 feet per second. After a minute, the fireball has cooled to such an extent that it no longer emits visible radiation. It has then risen roughly 4.5 miles from the point of burst.
THE RADIOACTIVE CLOUD
2.06 While the fireball is still luminous, the temperature, in the interior at least, is so high that all the weapon materials are in the form of vapor. This includes the radioactive fission products, uranium (or plutonium) that has escaped fission, and the weapon casing (and other) materials. As the fireball increases in size and cools, the vapors condense to form a cloud containing solid particles of the weapon debris, as well as many small drops of water derived from the air sucked into the rising fireball.
2.07 Quite early in the ascent of the fireball, cooling of the outside by radiation and the drag of the air through which it rises frequently bring about a change in shape. The roughly spherical form becomes a toroid (or doughnut), although this shape and its associated motion are often soon hidden by the radioactive cloud and debris. As it ascends, the toroid undergoes a violent, internal circulatory motion as shown in Fig. 2.07a. The formation of the toroid is usually observed in the lower part of the visible cloud, as may be seen in the lighter, i.e., more luminous, portion of Fig. 2.07b. The circulation entrains more air through the bottom of the toroid, thereby cooling the cloud and dissipating the energy contained in the fireball. As a result, the toroidal motion slows and may stop completely as the cloud rises toward its maximum height.
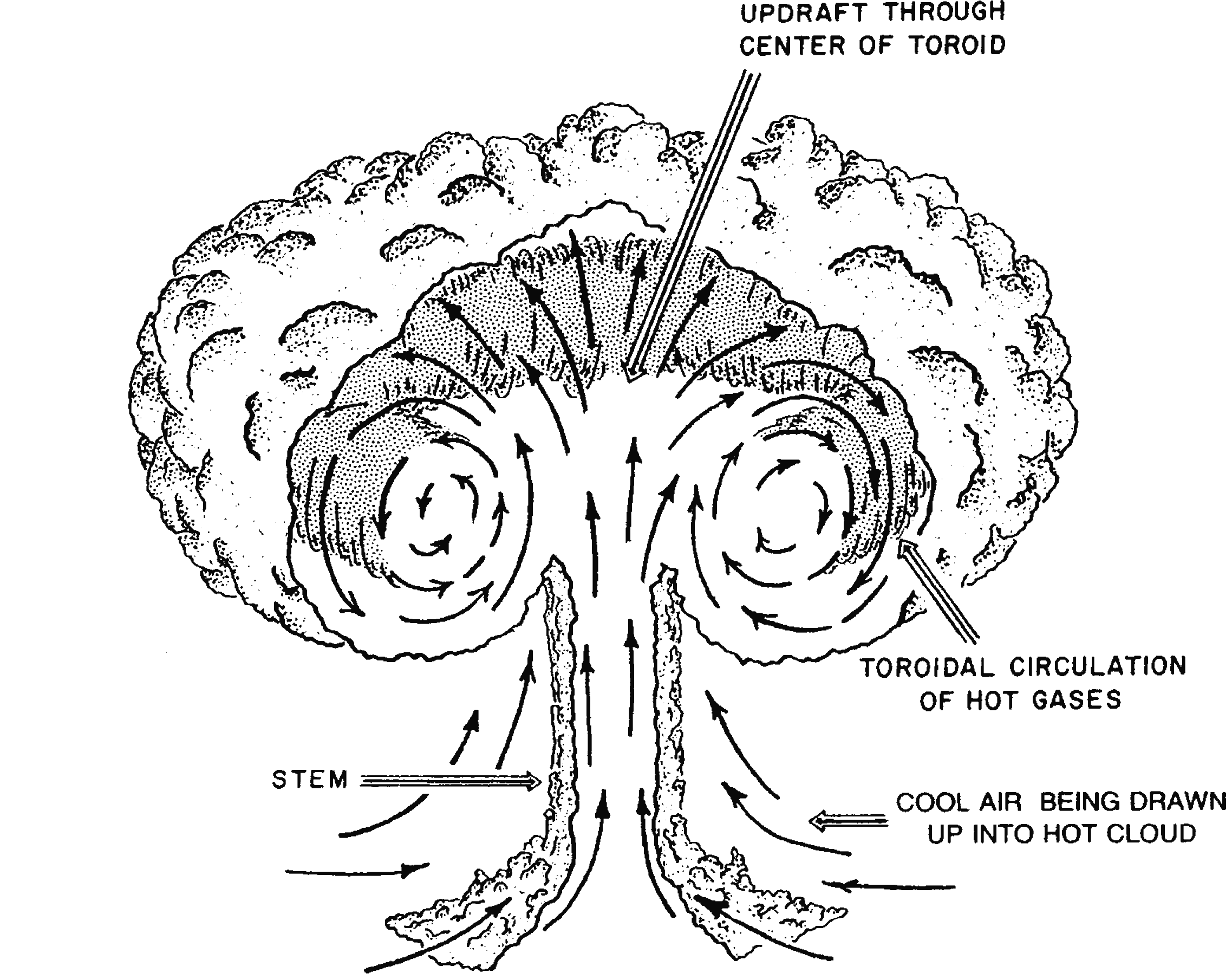
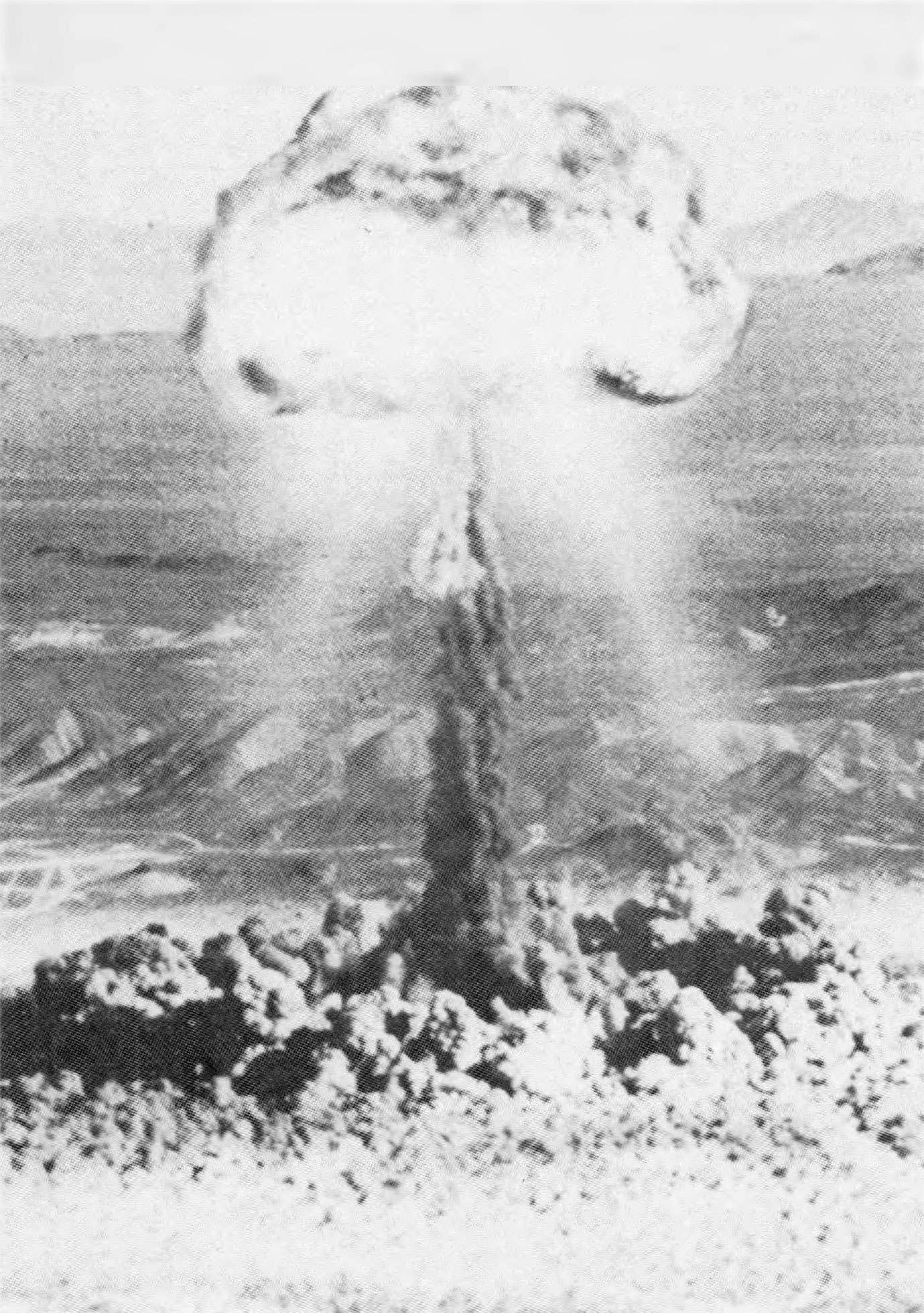
2.08 The color of the radioactive cloud is initially red or reddish brown, due to the presence of various colored compounds (nitrous acid and oxides of nitrogen) at the surface of the fireball. These result from chemical interaction of nitrogen, oxygen, and water vapor in the air at the existing high temperatures and under the influence of the nuclear radiations. As the fireball cools and condensation occurs, the color of the cloud changes to white, mainly due to the water droplets as in an ordinary cloud.
2.09 Depending on the height of burst of the nuclear weapon and the nature of the terrain below, a strong updraft with inflowing winds, called “afterwinds,” is produced in the immediate vicinity. These afterwinds can cause varying amounts of dirt and debris to be sucked up from the earth’s surface into the radioactive cloud (Fig. 2.07b).
2.10 In an air burst with a moderate (or small) amount of dirt and debris drawn up into the cloud, only a relatively small proportion of the dirt particles become contaminated with radioactivity. This is because the particles do not mix intimately with the weapon residues in the cloud at the time when the fission products are still vaporized and about to condense. For a burst near the land surface, however, large quantities of dirt and other debris are drawn into the cloud at early times. Good mixing then occurs during the initial phases of cloud formation and growth. Consequently, when the vaporized fission products condense they do so on the foreign matter, thus forming highly radioactive particles (§ 2.23).
2.11 At first the rising mass of weapon residues carries the particles upward, but after a time they begin to fall slowly under the influence of gravity. at rates dependent upon their size. Consequently, a lengthening (and widening) column of cloud (or smoke) is produced. This cloud consists chiefly of very small particles of radioactive fission products and weapon residues, water droplets, and larger particles of dirt and debris carried up by the afterwinds.
2.12 The speed with which the top of the radioactive cloud continues to ascend depends on the meteorological conditions as well as on the energy yield of the weapon. An approximate indication of the rate of rise of the cloud from a 1-megaton explosion is given by the results in Table 2.12 and the curve in Fig. 2 .12. Thus, in general, the cloud will have attained a height of 3 miles in 30 seconds and 5 miles in about a minute. The average rate of rise during the first minute or so is nearly 300 miles per hour (440 feet per second). These values should. be regarded as rough averages only, and large deviations may be expected in different circumstances (see also Figs. 10.158a, b, c).
Height (miles) |
Time (minutes) |
Rate of Rise (miles per hour) |
---|---|---|
2 | 0.3 | 330 |
4 | 0.7 | 270 |
6 | 1.1 | 220 |
10 | 2.5 | 140 |
12 | 3.8 | 27 |
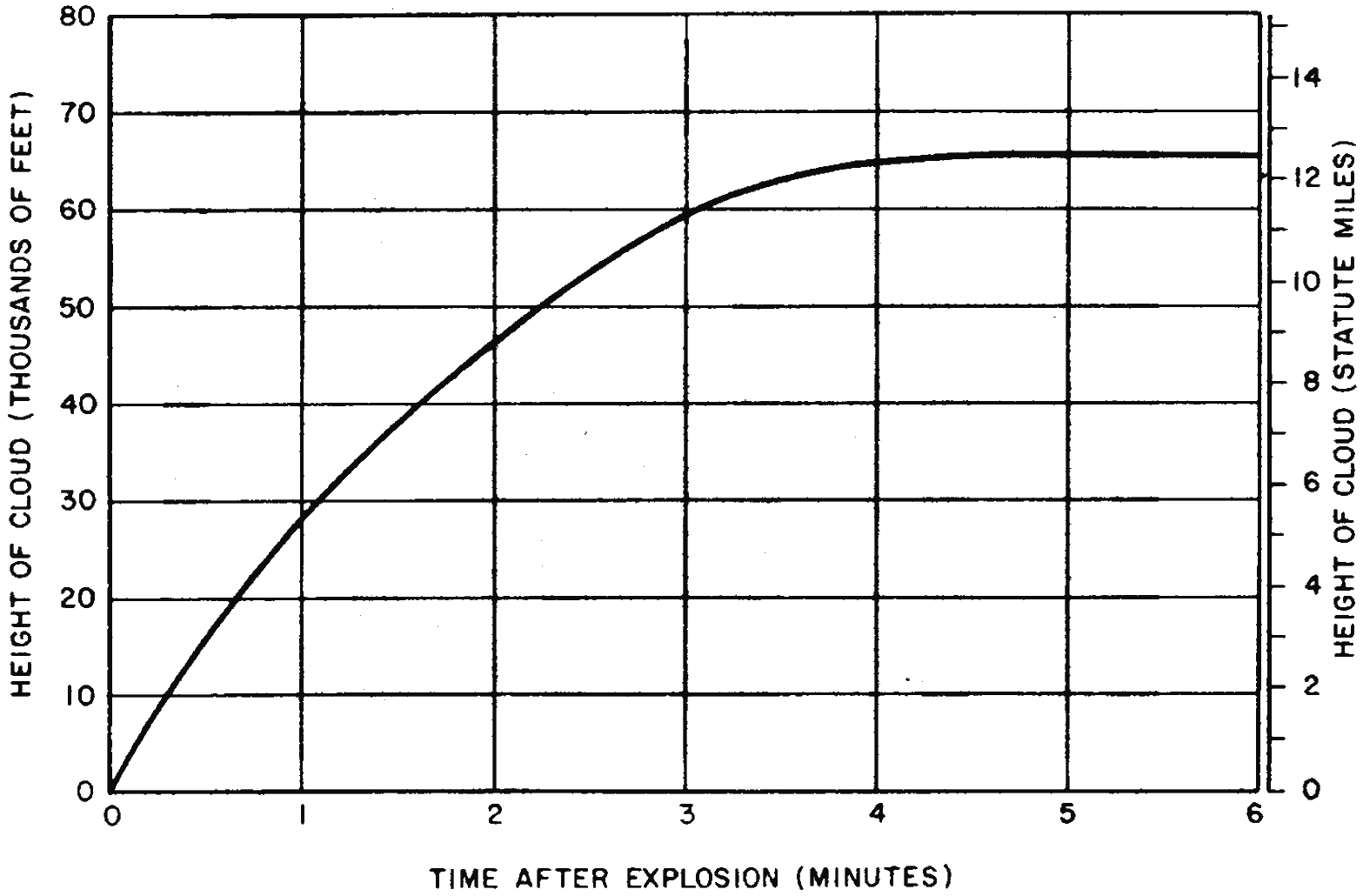
2.13 The eventual height reached by the radioactive cloud depends upon the heat energy of the weapon, and upon the atmospheric conditions, e.g., moisture content and stability. The greater the amount of heat generated the greater will be the upward thrust due to buoyancy and so the greater will be the distance the cloud ascends. The maximum height attained by the radioactive cloud is strongly influenced by the tropopause, i.e., the boundary between the troposphere below and the stratosphere above, assuming that the cloud attains the height of the troposphere.2
2.14 When the cloud reaches the tropopause, there is a tendency for it to spread out laterally, i.e., sideways. But if sufficient energy remains in the radioactive cloud at this height, a portion of it will penetrate the tropopause and ascend into the more stable air of the stratosphere.
2.15 The cloud attains its maximum height after about 10 minutes and is then said to be “stabilized.” It continues to grow laterally, however, to produce the characteristic mushroom shape (Fig. 2.15). The cloud may continue to be visible for about an hour or more before being dispersed by the winds into the surrounding atmosphere where it merges with natural clouds in the sky.
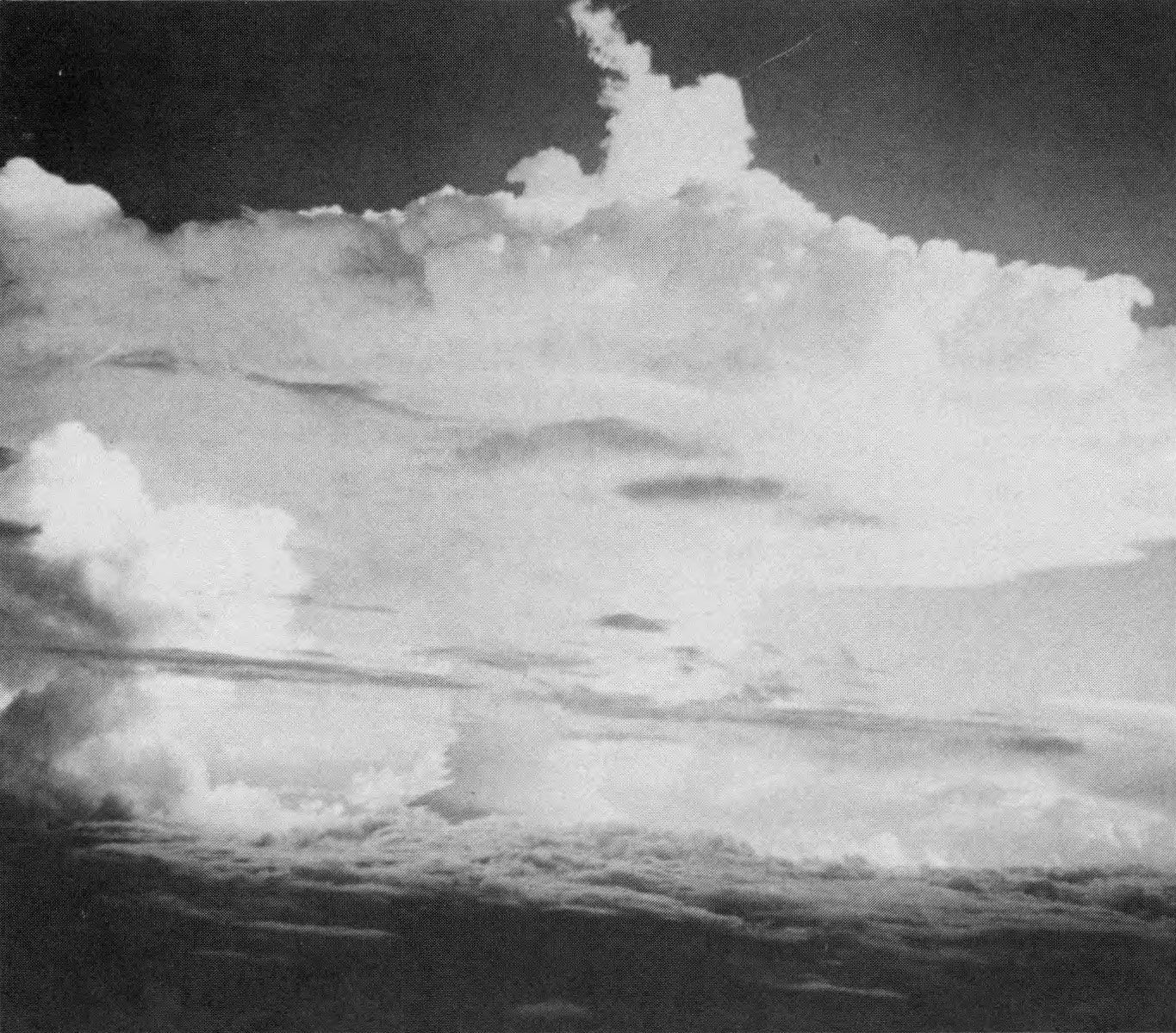
2.16 The dimensions of the stabilized cloud formed in a nuclear explosion depend on the meteorological conditions, which vary with time and place. Approximate average values of cloud height and radius (at about 10 minutes after the explosion), attained in land surface or low air bursts, for conditions most likely to be encountered in the continental United States, are given in Fig. 2.16 as a function of the energy yield of the explosion. The flattening of the height curve in the range of about 20- to 100-kilotons TNT equivalent is due to the effect of the tropopause in slowing down the cloud rise. For yields below about 15 kilotons the heights indicated are distances above the burst point but for higher yields the values are above sea level. For land surface bursts, the maximum cloud height is somewhat less than given by Fig. 2.16 because of the mass of dirt and debris carried aloft by the explosion.
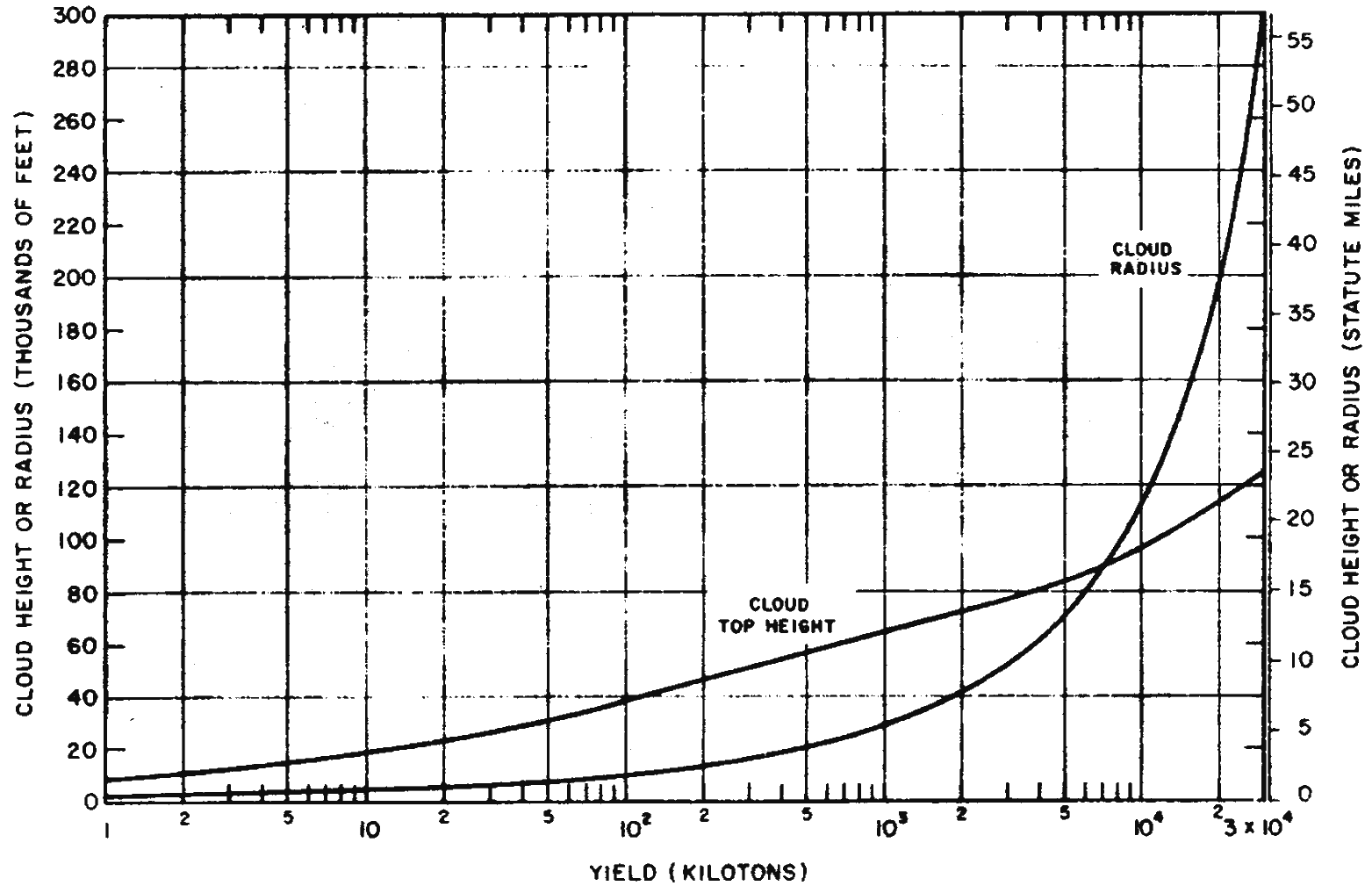
2.17 For yields below about 20 kilotons, the radius of the stem of the mushroom cloud is about half the cloud radius. With increasing yield, however, the ratio of these dimensions decreases, and for yields in the megaton range the stem may be only one-fifth to one-tenth as wide as the cloud. For clouds which do not penetrate the tropopause the base of the mushroom head is, very roughly, at about one-half the altitude of the top. For higher yields, the broad base will probably be in the vicinity of the tropopause. There is a change in cloud shape in going from the kiloton to the megaton range. A typical cloud from a 10-kiloton air burst would reach a height of 19,000 feet with the base at about 10,000 feet; the horizontal extent would also be roughly 10,000 feet. For an explosion in the megaton range, however, the horizontal dimensions are greater than the total height (cf. Fig. 2.16).
CHARACTERISTICS OF A SURFACE BURST
2.18 Since many of the phenomena and effects of a nuclear explosion occurring on or near the earth’s surface are similar to those associated with an air burst, it is convenient before proceeding further to refer to some of the special characteristics of a surface burst. In such a burst, the fireball in its rapid initial growth, abuts (or touches) the surface of the earth (Fig. 2.18a). Because of the intense heat, some of the rock, soil, and other material in the area is vaporized and taken into the fireball. Additional material is melted, either completely or on its surface, and the strong afterwinds cause large amounts of dirt, dust, and other particles to be sucked up as the fireball rises (Fig. 2.18b).
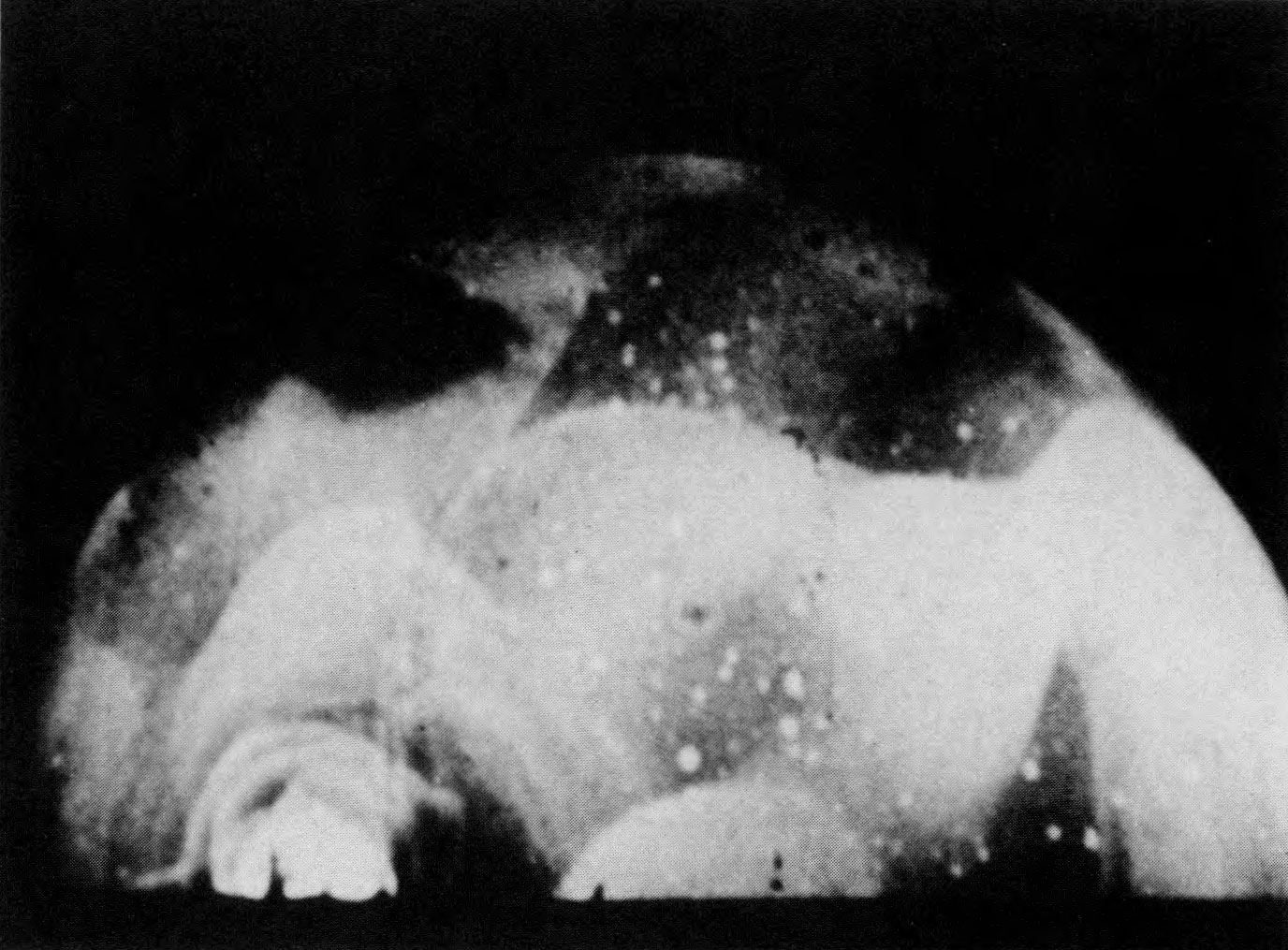
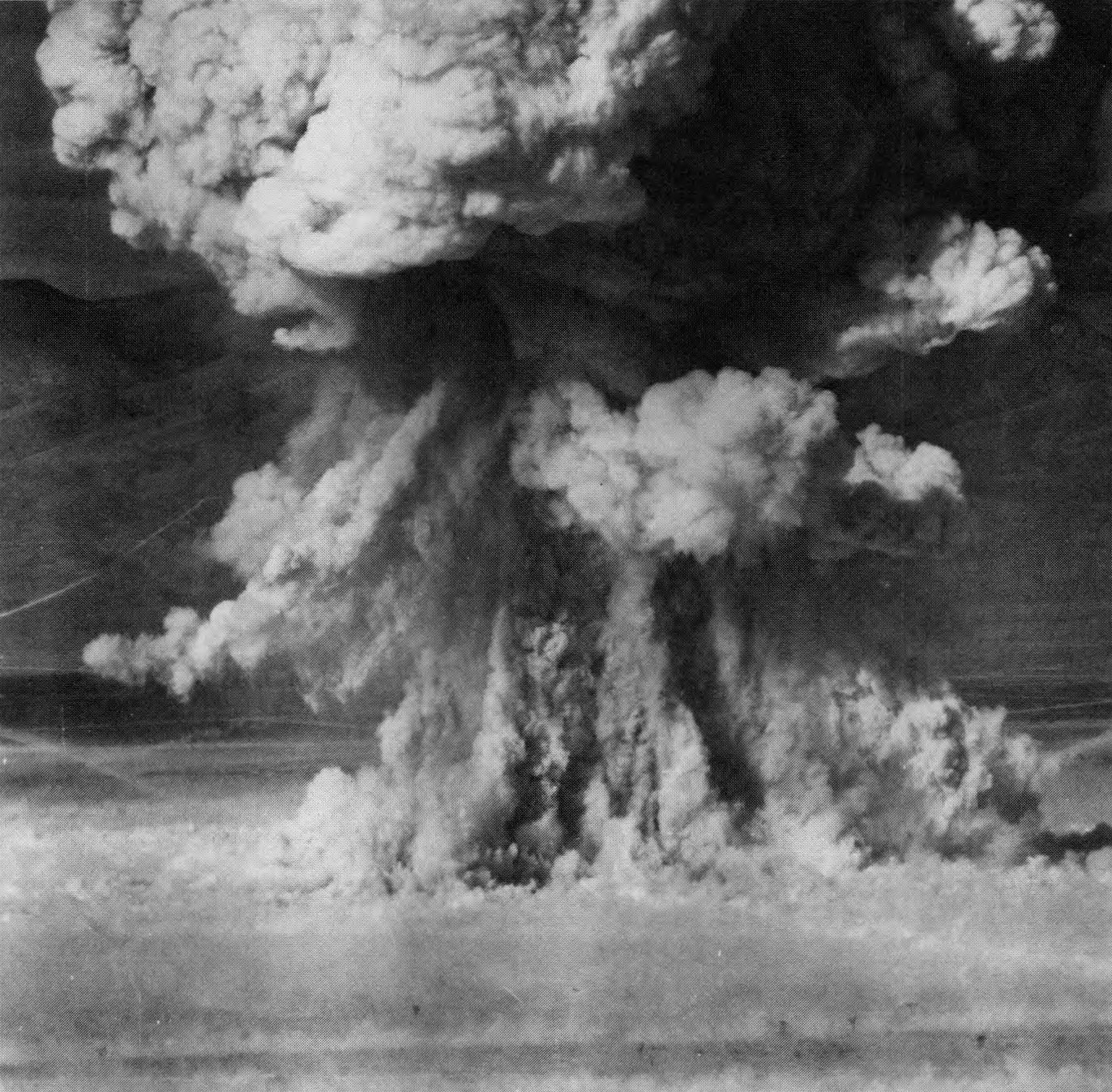
2.19 An important difference between a surface burst and an air burst is, consequently, that in the surface burst the radioactive cloud is much more heavily loaded with debris. This consists of particles ranging in size from the very small ones produced by condensation as the fireball cools to the much larger debris particles which have been raised by the afterwinds. The exact composition of the cloud will, of course, depend on the nature of the surface materials and the extent of their contact with the fireball.
2.20 For a surface burst associated with a moderate amount of debris, such as has been the case in several test explosions in which the weapons were detonated near the ground, the rate of rise of the cloud is much the same as given earlier for an air burst (Table 2.12). The radioactive cloud reaches a height of several miles before spreading out abruptly into a mushroom shape.
2.21 When the fireball touches the earth’s surface, a crater is formed as a result of the vaporization of dirt and other material and the removal of soil, etc., by the blast wave and winds accompanying the explosion. The size of the crater will vary with the height above the surface at which the weapon is exploded and with the character of the soil, as well as with the energy of the explosion. It is believed that for a 1 megaton weapon there would be no appreciable crater formation unless detonation occurs at an altitude of 450 feet or less.
2.22 If a nuclear weapon is exploded near a water surface, large amounts of water are vaporized and carried up into the radioactive cloud. When the cloud reaches high altitudes the vapor condenses to form water droplets, similar to those in an ordinary atmospheric cloud.
THE FALLOUT
2.23 In a surface burst, large quantities of earth or water enter the fireball at an early stage and are fused or vaporized. When sufficient cooling has occurred, the fission products and other radioactive residues become incorporated with the earth particles as a result of the condensation of vaporized fission products into fused particles of earth, etc. A small proportion of the solid particles formed upon further cooling are contaminated fairly uniformly throughout with the radioactive fission products and other weapon residues,3 but as a general rule the contamination is found mainly in a thin shell near the surface of the particle (§ 9.50). In water droplets, the small fission product particles occur at discrete points within the drops. As the violent disturbance due to the explosion subsides, the contaminated particles and droplets gradually descend to earth. This phenomenon is referred to as “fallout,” and the same name is applied to the particles themselves when they reach the ground. It is the fallout, with its associated radioactivity which decays over a long period of time, that is the main source of the residual nuclear radiation referred to in the preceding chapter.
2.24 The extent and nature of the fallout can range between wide extremes. The actual situation is determined by a combination of circumstances associated with the energy yield and design of the weapon, the height of the explosion, the nature of the surface beneath the point of burst, and the meteorological conditions. In an air burst, for example, occurring at an appreciable distance above the earth’s surface, so that no large amounts of surface materials are sucked into the cloud, the contaminated particles become widely dispersed. The magnitude of the hazard from fallout will then be far less than if the explosion were a surface burst. Thus at Hiroshima (height of burst 1670 feet, yield about 12.5 kilotons) and Nagasaki (height of burst 1,640 feet, yield about 22 kilotons) injuries due to fallout were completely absent.
2.25 On the other hand, a nuclear explosion occurring at or near the earth’s surface can result in severe contamination by the radioactive fallout. From the 15-megaton thermonuclear device tested at Bikini Atoll on March 1, 1954-the BRAVO shot of Operation CASTLE-the fallout caused substantial contamination over an area of more than 7,000 square miles. The contaminated region was roughly cigar shaped and extended more than 20 statute miles upwind and over 350 miles downwind. The width in the crosswind direction was variable, the maximum being over 60 miles (§ 9.104).
2.26 The meteorological conditions which determine the shape, extent, and location of the fallout pattern from a nuclear explosion are the height of the tropopause, atmospheric winds, and the occurrence of precipitation. For a given explosion energy yield, type of burst, and tropopause height, the fallout pattern is affected mainly by the directions and speeds of the winds over the fallout area, from the earth’s surface to the top of the stabilized cloud, which may be as high as 100,000 feet. Furthermore, variations in the winds, from the time of burst until the particles reach the ground, perhaps several hours later, affect the fallout pattern following a nuclear explosion (see Chapter IX).
2.27 It should be understood that fallout is a gradual phenomenon extending over a period of time. In the BRAVO explosion, for example, about 10 hours elapsed before the contaminated particles began to fall at the extremities of the 7,000 square mile area. By that time, the radioactive cloud had thinned out to such an extent that it was no longer visible. This brings up the important fact that fallout can occur even when the cloud cannot be seen. Nevertheless, the area of contamination which presents the most serious hazard generally results from the fallout of visible particles. The sizes of these particles range from that of fine sand, i.e., approximately 100 micrometers4 in diameter, or smaller, in the more distant portions of the fallout area, to pieces about the size of a marble, i.e., roughly I cm (0.4 inch) in diameter, and even larger close to the burst point.
2.28 Particles in this size range arrive on the ground within one day after the explosion, and will not have traveled too far, e.g., up to a few hundred miles, from the region of the shot, depending on the wind. Thus, the fallout pattern from particles of visible size is established within about 24 hours after the burst. This is referred to as “early” fallout, also sometimes called “local” or “close-in” fallout. In addition, there is the deposition of very small particles which descend very slowly over large areas of the earth’s surface. This is the “delayed” (or “worldwide”) fallout, to which residues from nuclear explosions of various types-air, high-altitude, surface, and shallow subsurface-may contribute (see Chapter IX).
2.29 Although the test of March 1, 1954 produced the most extensive early fallout yet recorded, it should be pointed out that the phenomenon was not necessarily characteristic of (nor restricted to) thermonuclear explosions. It is very probable that if the same device had been detonated at an appreciable distance above the coral island, so that the large fireball did not touch the surface of the ground, the early fallout would have been of insignificant proportions.
2.30 The general term “scavenging” is used to describe various processes resulting in the removal of radioactivity from the cloud and its deposition on the earth. One of these processes arises from the entrainment in the cloud of quantities of dirt and debris sucked up in a surface (or near-surface) nuclear burst. The condensation of the fission-product and other radioactive vapors on the particles and their subsequent relatively rapid fall to earth leads to a certain degree of scavenging.
2.31 Another scavenging process, which can occur at any time in the history of the radioactive cloud, is that due to rain falling through the weapon debris and carrying contaminated particles down with it. This is one mechanism for the production of “hot spots,” i.e., areas on the ground of much higher activity than the surroundings, in both early and delayed fallout patterns. Since rains (other than thundershowers) generally originate from atmospheric clouds whose tops are between about 10,000 and 30,000 feet altitude, it is only below this region that scavenging by rain is likely to take place. Another effect that rain may have if it occurs either during or after the deposition of the fallout is to wash radioactive debris over the surface of the ground. This may result in cleansing some areas and reducing their activity while causing hot spots in other (lower) areas.
THE BLAST WAVE
2.32 At a fraction of a second after a nuclear explosion, a high-pressure wave develops and moves outward from the fireball (Fig. 2.32). This is the shock wave or blast wave, mentioned in§ 1.01 and to be considered subsequently in more detail, which is the cause of much destruction accompanying an air burst. The front of the blast wave, i.e., the shock front, travels rapidly away from the fireball, behaving like a moving wall of highly compressed air. After the lapse of 10 seconds, when the fireball of a 1-megaton nuclear weapon has attained its maximum size (5,700 feet across), the shock front is some 3 miles farther ahead. At 50 seconds after the explosion, when the fireball is no longer visible, the blast wave has traveled about 12 miles. It is then moving at about 1,150 feet per second, which is slightly faster than the speed of sound at sea level.
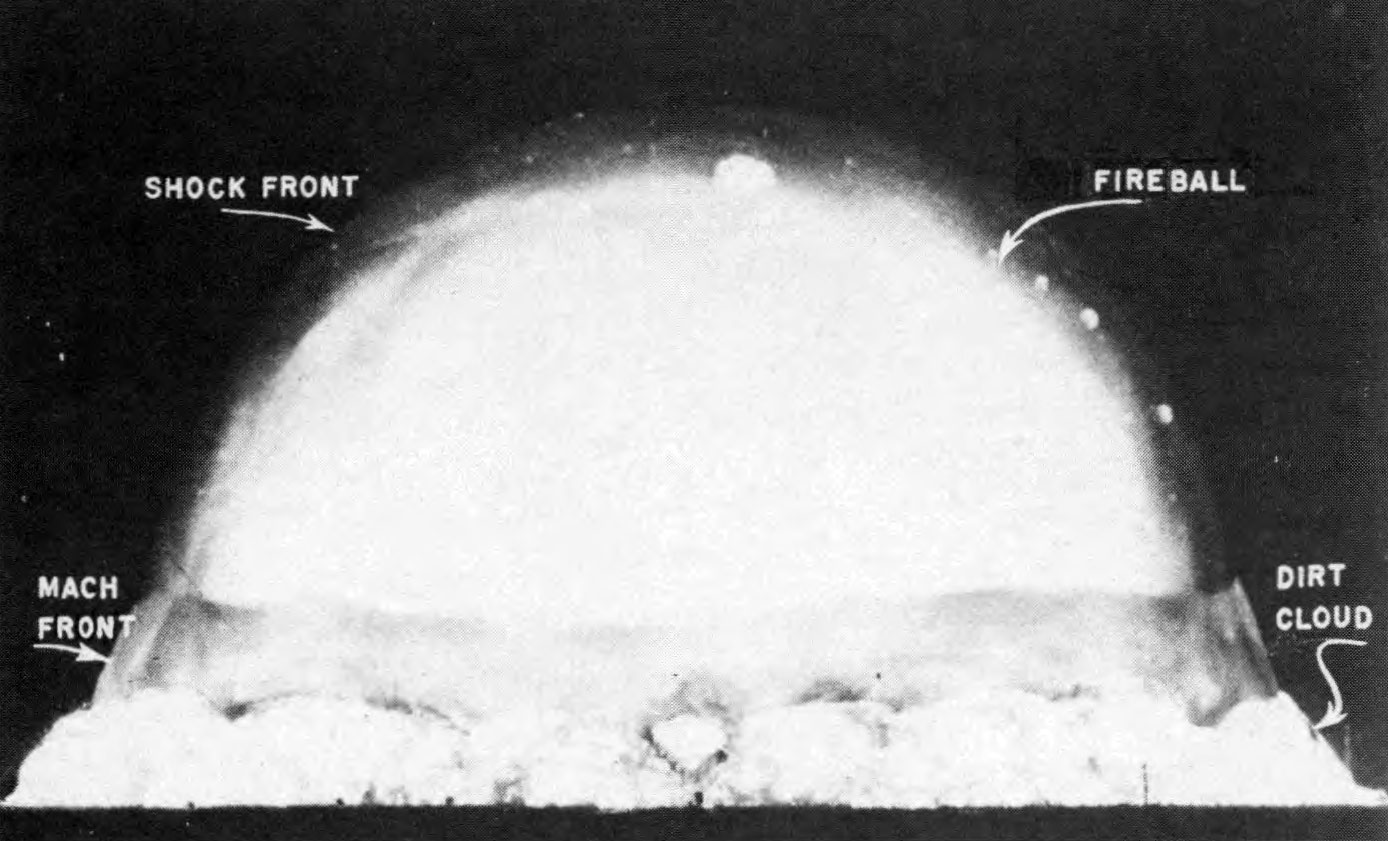
2.33 When the blast wave strikes the surface of the earth, it is reflected back, similar to a sound wave producing an echo. This reflected blast wave, like the original (or direct) wave, is also capable of causing material damage. At a certain region on the surface, the position of which depends chiefly on the height of the burst and the energy of the explosion, the direct and reflected wave fronts merge. This merging phenomenon is called the “Mach effect.” The “overpressure,” i.e. , the pressure in excess of the normal atmospheric value, at the front of the Mach wave is generally about twice as great as that at the direct blast wave front.
2.34 For an air burst of a 1-megaton nuclear weapon at an altitude of 6,500 feet, the Mach effect will begin approximately 4.5 seconds after the explosion, in a rough circle at a radius of 1.3 miles from ground zero.5 The overpressure on the ground at the blast wave front at this time is about 20 pounds per square inch, so that the total air pressure is more than double the normal atmospheric pressure.6
2.35 At first the height of the Mach front is small, but as the blast wave front continues to move outward, the height increases steadily. At the same time, however, the overpressure, like that in the original (or direct) wave, decreases correspondingly because of the continuous loss of energy and the ever-increasing area of the advancing front. After the lapse of about 40 seconds, when the Mach front from a 1-megaton nuclear weapon is 10 miles from ground zero, the overpressure will have decreased to roughly 1 pound per square inch.
2.36 The distance from ground zero at which the Mach effect commences varies with the height of burst. Thus, as seen in Fig. 2.32, in the low-altitude (100 feet) detonation at the TRINITY (Alamogordo) test, the Mach front was apparent when the direct shock front had advanced a short distance from the fireball. At the other extreme, in a very high air burst there might be no detectable Mach effect. (The TRINITY test, conducted on July 16, 1945 near Alamogordo, New Mexico, was the first test of a nuclear (implosion) weapon; the yield was estimated to be about 19 kilotons.)
2.37 Strong transient winds are associated with the passage of the shock (and Mach) front. These blast winds (§ 3.07) are very much stronger than the ground wind (or afterwind) due to the updraft caused by the rising fireball (§ 2.09) which occurs at a later time. The blast winds may have peak velocities of several hundred miles an hour fairly near to ground zero; even at more than 6 miles from the explosion of a 1-megaton nuclear weapon, the peak velocity will be greater than 70 miles per hour. It is evident that such strong winds can contribute greatly to the blast damage resulting from the explosion of a nuclear weapon.
THERMAL RADIATION FROM AN AIR BURST
2.38 Immediately after the explosion, the weapon residues emit the primary thermal radiation (§ 1.77). Because of the very high temperature, much of this is in the form of X rays which are absorbed within a layer of a few feet of air; the energy is then reemitted from the fireball as (secondary) thermal radiation of longer wavelength, consisting of ultraviolet, visible, and infrared rays. Because of certain phenomena occurring in the fireball (see § 2.106 et seq.), the surface temperature undergoes a curious change. The temperature of the interior falls steadily, but the apparent surface temperature of the fireball decreases more rapidly for a small fraction of a second. Then, the apparent surface temperature increases again for a somewhat longer time, after which it falls continuously (see Fig. 2.123). In other words, there are effectively two surface-temperature pulses; the first is of very short duration, whereas the second lasts for a much longer time. The behavior is quite general for air (and surface) bursts, although the duration times of the pulses increase with the energy yield of the explosion.
2.39 Corresponding to the two surface-temperature pulses, there are two pulses of emission of thermal radiation from the fireball (Fig. 2.39). In the first pulse, which lasts about a tenth of a second for a 1-megaton explosion, the surface temperatures are mostly very high. As a result, much of the radiation emitted by the fireball during this pulse is in the ultraviolet region. Although ultraviolet radiation can cause skin burns, in most circumstances following an ordinary air burst the first pulse of thermal radiation is not a significant hazard in this respect, for several reasons. In the first place, only about 1 percent of the thermal radiation appears in the initial pulse because of its short duration. Second, the ultraviolet rays are readily attenuated by the intervening air, so that the dose delivered at a distance from the explosion may be comparatively small. Furthermore, it appears that the ultraviolet radiation from the first pulse could cause significant effects on the human skin only within ranges at which other thermal radiation effects are much more serious. It should be mentioned, however, that although the first radiation pulse may be disregarded as a source of skin burns, it is capable of producing permanent or temporary effects on the eyes, especially of individuals who happen to be looking in the direction of the explosion.
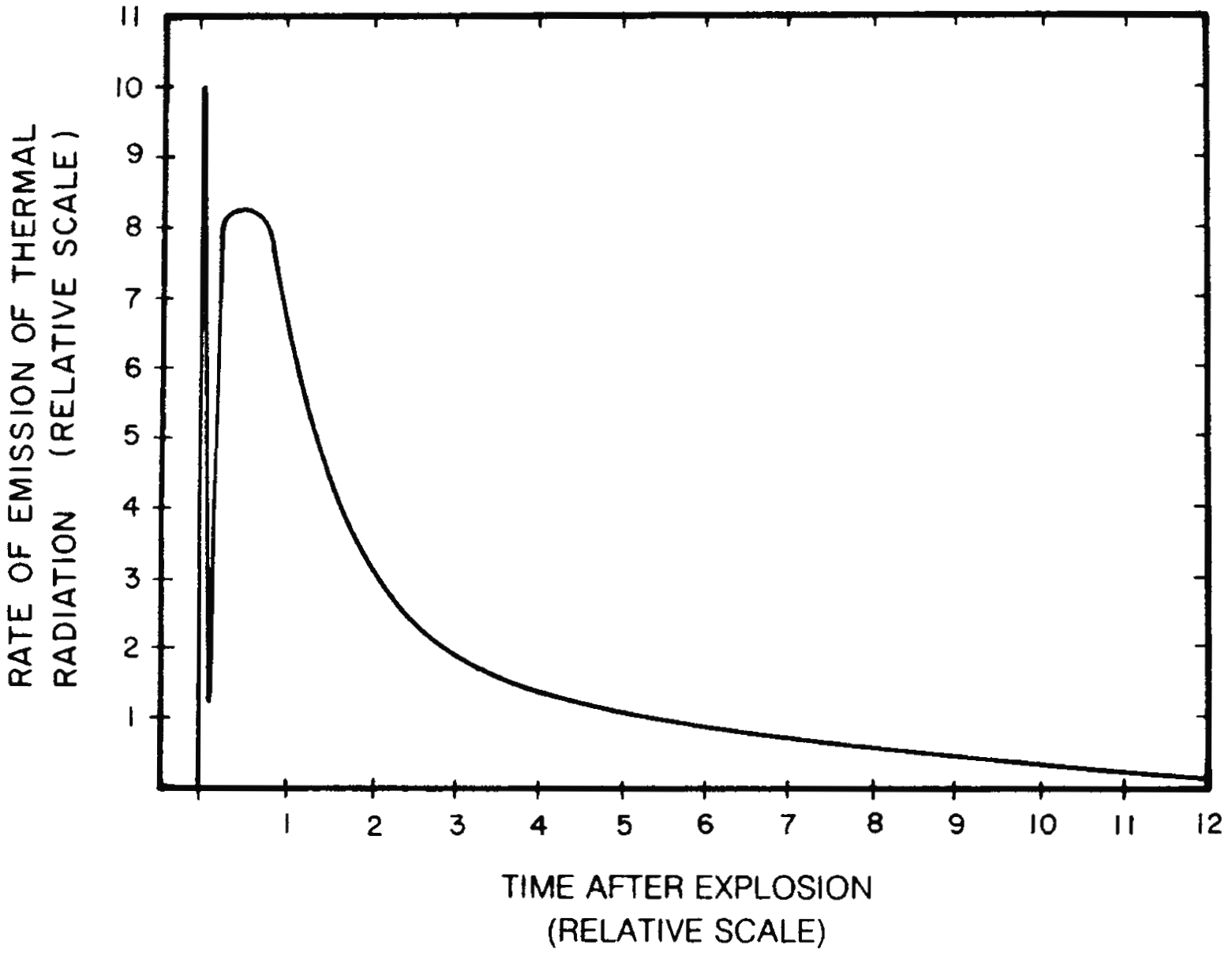
2.40 In contrast to the first pulse, the second radiation pulse may last for several seconds, e.g., about 10 seconds for a 1-megaton explosion; it carries about 99 percent of the total thermal radiation energy. Since the temperatures are lower than in the first pulse, most of the rays reaching the earth consist of visible and infrared (invisible) light. It is this radiation which is the main cause of skin burns of various degrees suffered by exposed individuals up to 12 miles or more, and of eye effects at even greater distances, from the explosion of a 1-megaton weapon. For weapons of higher energy, the effective damage range is greater, as will be explained in Chapter VII. The radiation from the second pulse can also cause fires to start under suitable conditions.
INITIAL NUCLEAR RADIATION FROM AN AIR BURST
2.41 As stated in Chapter I, the explosion of a nuclear weapon is associated with the emission of various nuclear radiations, consisting of neutrons, gamma rays, and alpha and beta particles. Essentially all the neutrons and part of the gamma rays are emitted in the actual fission process. These are referred to as the “prompt nuclear radiations” because they are produced simultaneously with the nuclear explosion. Some of the neutrons liberated in fission are immediately captured and others undergo “scattering collisions” with various nuclei present in the weapon. These processes are frequently accompanied by the instantaneous emission of gamma rays. In addition, many of the escaping neutrons undergo similar interactions with atomic nuclei of the air, thus forming an extended source of gamma rays around the burst point. The remainder of the gamma rays and the beta particles are liberated over a period of time as the fission products undergo radioactive decay. The alpha particles are expelled, in an analogous manner, as a result of the decay of the uranium (or plutonium) which has escaped fission in the weapon.
2.42 The initial nuclear radiation is generally defined as that emitted from both the fireball and the radioactive cloud within the first minute after the explosion. It includes neutrons and gamma rays given off almost instantaneously, as well as the gamma rays emitted by the fission products and other radioactive species in the rising cloud. It should be noted that the alpha and beta particles present in the initial radiation have not been considered. This is because they are so easily absorbed that they will not reach more than a few yards, at most, from the radioactive cloud.
2.43 The somewhat arbitrary time period of 1 minute for the duration of the initial nuclear radiations was originally based upon the following considerations. As a consequence of attenuation by the air, the effective range of the fission gamma rays and of those from the fission products from a 20-kiloton explosion is very roughly 2 miles. In other words, gamma rays originating from such a source at an altitude of over 2 miles can be ignored, as far as their effect at the earth’s surface is concerned. Thus, when the radioactive cloud has reached a height of 2 miles, the effects of the initial nuclear radiations are no longer significant. Since it takes roughly a minute for the cloud to rise this distance, the initial nuclear radiation was defined as that emitted in the first minute after the explosion.
2.44 The foregoing arguments are based on the characteristics of a 20-kiloton nuclear weapon. For a detonation of higher energy, the maximum distance over which the gamma rays are effective will be larger than given above. However, at the same time, there is an increase in the rate at which the cloud rises. Similarly for a weapon of lower energy, the effective distance is less, but so also is the rate of ascent of the cloud. The period over which the initial nuclear radiation extends may consequently be taken to be approximately the same, namely, 1 minute, irrespective of the energy release of the explosion.
2.45 Neutrons are the only significant nuclear radiations produced directly in the thermonuclear reactions mentioned in § 1.69. Alpha particles (helium nuclei) are also formed, but they do not travel very far from the explosion. Some of the neutrons will escape but others will be captured by the various nuclei present in the exploding weapon. Those neutrons absorbed by fissionable species may lead to the liberation of more neutrons as well as to the emission of gamma rays. In addition, the capture of neutrons in nonfission reactions is usually accompanied by gamma rays. It is seen, therefore, that the initial radiations from an explosion in which both fission and fusion (thermonuclear) processes occur consist essentially of neutrons and gamma rays. The relative proportions of these two radiations may be somewhat different than for a weapon in which all the energy release is due to fission, but for present purposes the difference may be disregarded.
THE ELECTROMAGNETIC PULSE
2.46 If a detonation occurs at or near the earth’s surface, the EMP phenomenon referred to in § 1.38 produces intense electric and magnetic fields which may extend to distances up to several miles, depending on the weapon yield. The close-in region near the burst point is highly ionized and large electric currents flow in the air and the ground, producing a pulse of electromagnetic radiation. Beyond this close-in region the electromagnetic field strength, as measured on (or near) the ground, drops sharply and then more slowly with increasing distance from the explosion. The intense fields may damage unprotected electrical and electronic equipment at distances exceeding those at which significant air blast damage may occur, especially for weapons of low yield (see Chapter XI).
OTHER NUCLEAR EXPLOSION PHENOMENA
2.47 There are a number of interesting phenomena associated with a nuclear air burst that are worth mentioning although they have no connection with the destructive or other harmful effects of the explosion. Soon after the detonation, a violet-colored glow may be observed, particularly at night or in dim daylight, at some distance from the fireball. This glow may persist for an appreciable length of time, being distinctly visible near the head of the radioactive cloud. It is believed to be the ultimate result of a complex series of processes initiated by the action of the various radiations on the nitrogen and oxygen of the air.
2.48 Another early phenomenon following a nuclear explosion in certain circumstances is the formation of a “condensation cloud.” This is sometimes called the Wilson cloud (or cloud-chamber effect) because it is the result of conditions analogous to those utilized by scientists in the Wilson cloud chamber. It will be seen in Chapter III that the passage of a high-pressure shock front in air is followed by a rarefaction (or suction) wave. During the compression (or blast) phase, the temperature of the air rises and during the decompression (or suction) phase it falls. For moderately low blast pressures, the temperature can drop below its original, preshock value, so that if the air contains a fair amount of water vapor, condensation accompanied by cloud formation will occur.
2.49 The condensation cloud which was observed in the ABLE Test at Bikini in 1946 is shown in Fig. 2.49. Since the device was detonated just above the surface of the lagoon, the air was nearly saturated with water vapor and the conditions were suitable for the production of a Wilson cloud. It can be seen from the photograph that the cloud formed some way ahead of the fireball. The reason is that the shock front must travel a considerable distance before the blast pressure has fallen sufficiently for a low temperature to be attained in the subsequent decompression phase. At the time the temperature has dropped to that required for condensation to occur, the blast wave front has moved still farther away, as is apparent in Fig. 2.49, where the disk-like formation on the surface of the water indicates the passage of the shock wave.
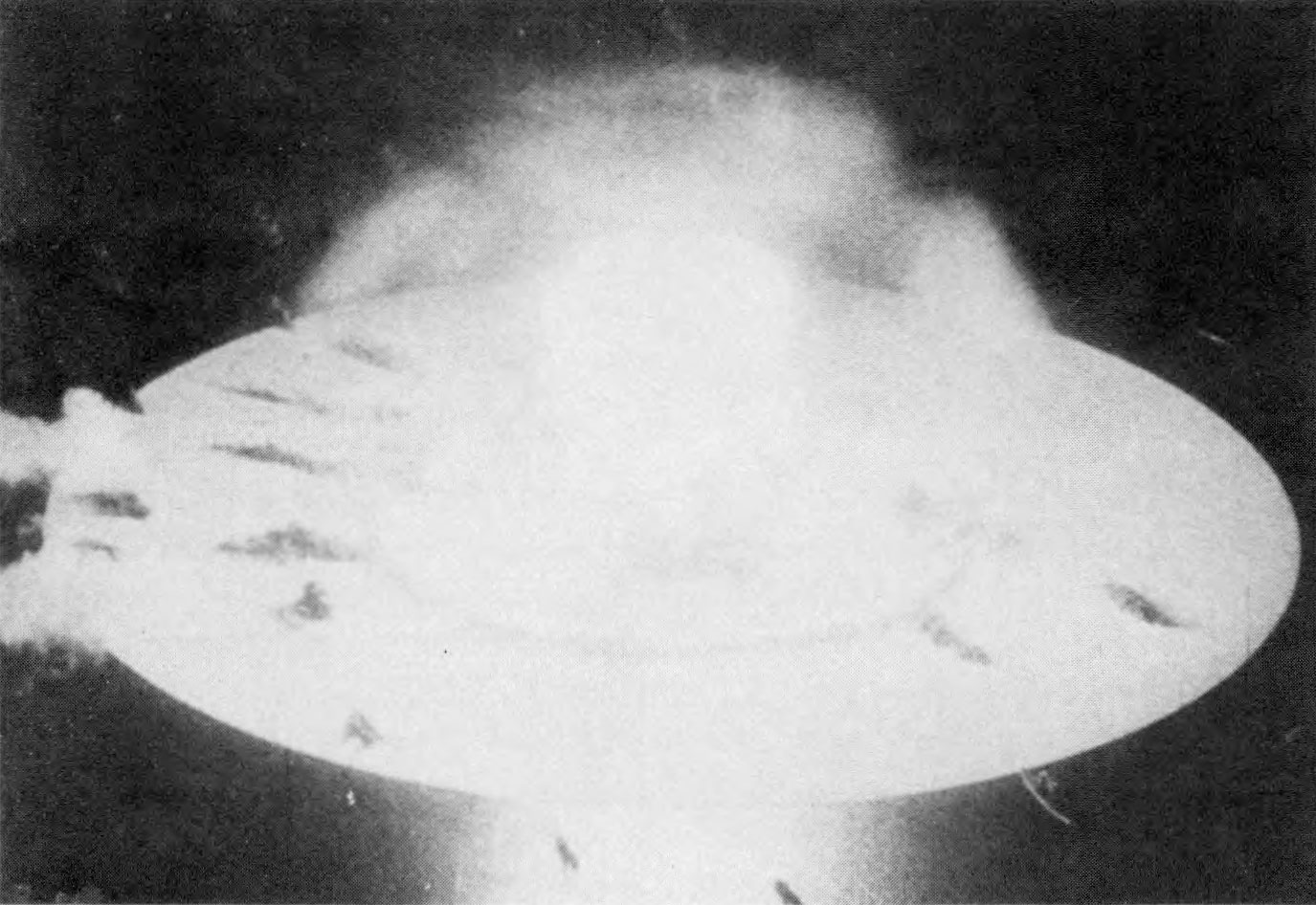
2.50 The relatively high humidity of the air makes the conditions for the formation of the condensation cloud most favorable in nuclear explosions occurring over (or under) water, as in the Bikini tests in 1946. The cloud commenced to form 1 to 2 seconds after the detonation, and it had dispersed completely within another second or so,as the air warmed up and the water droplets evaporated. The original dome-like cloud first changed to a ring shape, as seen in Fig. 2. 50, and then disappeared.
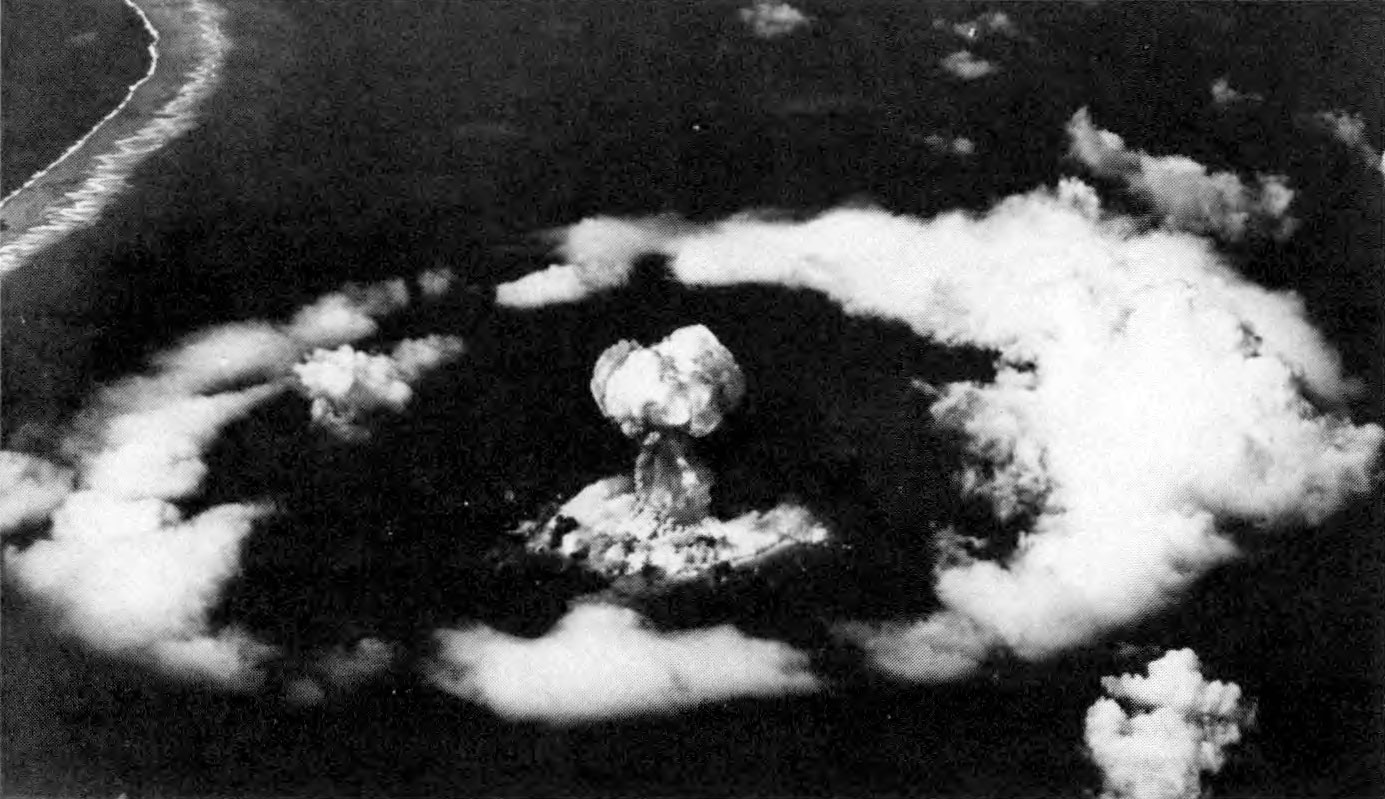
2.51 Since the Wilson condensation cloud forms after the fireball has emitted most of its thermal radiation, it has little influence on this radiation. It is true that fairly thick clouds, especially smoke clouds, can attenuate the thermal radiation reaching the earth from the fireball. However, apart from being formed at too late a stage, the condensation cloud is too tenuous to have any appreciable effect in this connection.
DESCRIPTION OF HIGH-ALTITUDE BURSTS
INTRODUCTION
2.52 Nuclear devices were exploded at high altitudes during the summer of 1958 as part of the HARDTACK test series in the Pacific Ocean and the ARGUS operation in the South Atlantic Ocean. Additional high-altitude nuclear tests were conducted during the FISHBOWL test series in 1962. In the HARDTACK series, two high-altitude bursts, with energy yields in the megaton range, were set off in the vicinity of Johnston Island, 700 miles southwest of Hawaii. The first device, named TEAK, was detonated on August 1, 1958 (Greenwich Civil Time) at an altitude of 252,000 feet, i.e., nearly 48 miles. The second, called ORANGE, was exploded at an altitude of 141,000 feet, i.e., nearly 27 miles, on August 12, 1958 (GCT). During the FISHBOWL series, a megaton and three submegaton devices were detonated at high altitudes in the vicinity of Johnston Island. The STARFISH PRIME device, with a yield of 1.4 megatons, was exploded at an altitude of about 248 miles on July 9, 1962 (GCT). The three submegaton devices, CHECKMATE, BLUEGILL TRIPLE PRIME, and KINGFISH, were detonated at altitudes of tens of miles on October 20, 1962, October 26, 1962, and November 1, 1962 (GCT), respectively.
2.53 The ARGUS operation was not intended as a test of nuclear weapons or their destructive effects. It was an experiment designed to provide information on the trapping of electrically charged particles in the earth’s magnetic field (§ 2.145). The operation consisted of three high-altitude nuclear detonations, each having a yield from 1 to 2 kilotons TNT equivalent. The burst altitudes were from about 125 to 300 miles.
HIGH-ALTITUDE BURST PHENOMENA
2.54 If a burst occurs in the altitude regime of roughly 10 to 50 miles, the explosion energy radiated as X rays will be deposited in the burst region, although over a much larger volume of air than at lower altitudes. In this manner, the ORANGE shot created a large fireball almost spherical in shape. In general, the fireball behavior was in agreement with the expected interactions of the various radiations and kinetic energy of the expanding weapon debris with the ambient air (§ 2.130 et seq.).
2.55 The mechanism of fireball formation changes appreciably at still higher burst altitude, since the X rays are able to penetrate to greater distances in the low-density air. Starting at an explosion altitude of about 50 miles, the interaction of the weapon debris energy with the atmosphere becomes the dominant mechanism for producing a fireball. Because the debris is highly ionize (§ 1.38), the earth’s magnetic field, i.e., the geomagnetic field, will influence the location and distribution of the late-time fireball from bursts above about 50 miles altitude.
2.56 The TEAK explosion was accompanied by a sharp and bright flash of light which was visible above the horizon from Hawaii, over 700 miles away. Because of the long range of the X rays in the low-density atmosphere in the immediate vicinity of the burst, the fireball grew very rapidly in size. In 0.3 second, its diameter was already 11 miles and it increased to 18 miles in 3.5 seconds. The fireball also ascended with great rapidity, the initial rate of rise being about a mile per second. Surrounding the fireball was a very large red luminous spherical wave, arising apparently from electronically excited oxygen atoms produced by a shock wave passing through the low-density air (Fig. 2.56).
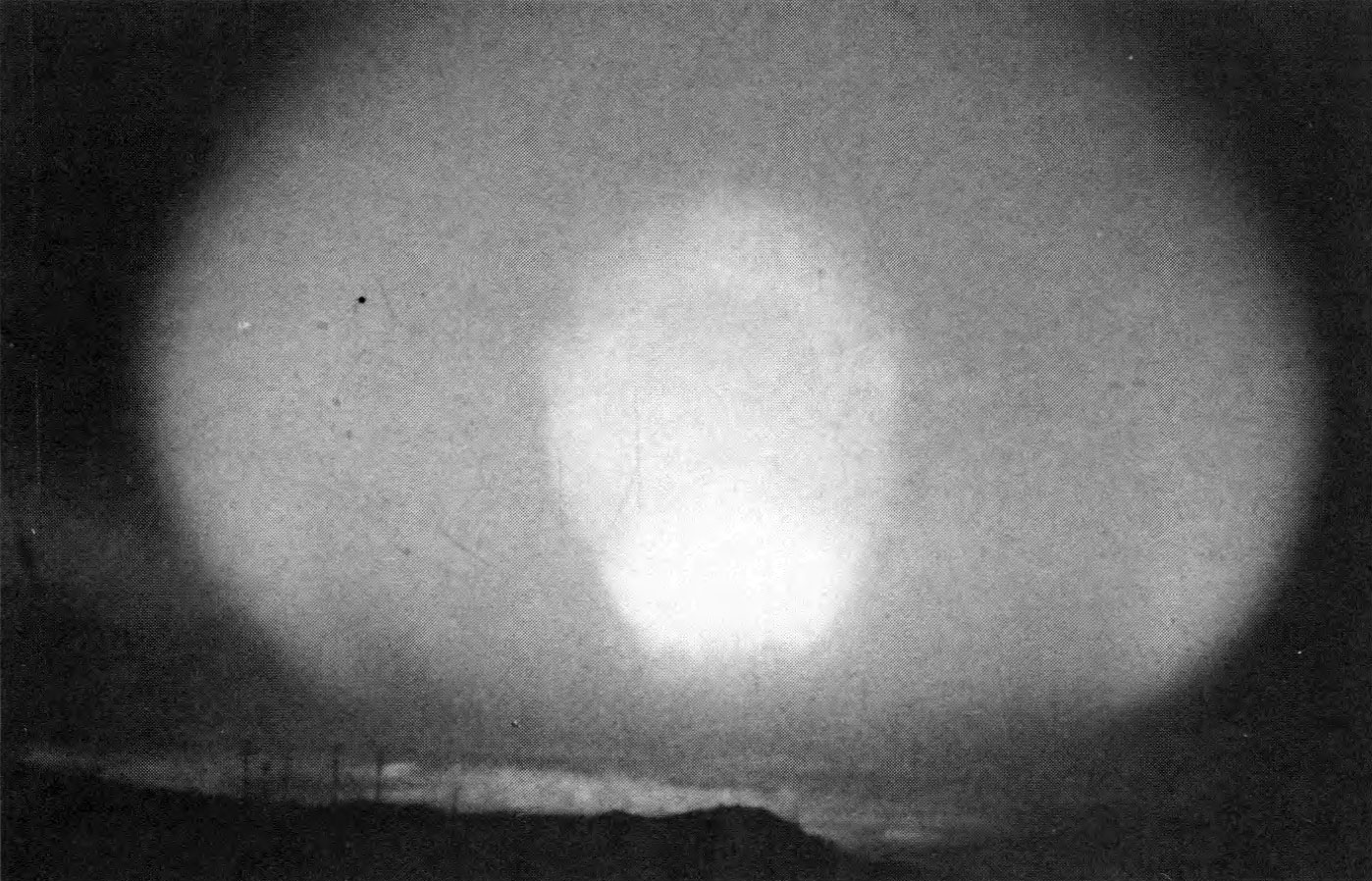
2.57 At about a minute or so after the detonation, the TEAK fireball had risen to a height of over 90 miles, and it was then directly (line-of-sight) visible from Hawaii. The rate of rise of the fireball was estimated to be some 3,300 feet per second and it was expanding horizontally at a rate of about 1,000 feet per second. The large red luminous sphere was observed for a few minutes; at roughly 6 minutes after the explosion it was nearly 600 miles in diameter.
2.58 The formation and growth of the fireball changes even more drastically as the explosion altitude increases above 65 miles. Because X rays can penetrate the low-density atmosphere to great distances before being absorbed, there is no local fireball. Below about 190 miles (depending on weapon yield), the energy initially appearing as the rapid outward motion of debris particles will still be deposited relatively locally, resulting in a highly heated and ionized region. The geomagnetic field plays an increasingly important role in controlling debris motion as the detonation altitude increases. Above about 200 miles, where the air density is very low, the geomagnetic field is the dominant factor in slowing the expansion of the ionized debris across the field lines. Upward and downward motion along the field lines, however, is not greatly affected (§ 10.64). When the debris is stopped by the atmosphere, at about 75 miles altitude, it may heat and ionize the air sufficiently to cause a visible region which will subsequently rise and expand. Such a phenomenon was observed following the STARFISH PRIME event.
2.59 A special feature of explosions at altitudes between about 20 and 50 miles is the extreme brightness of the fireball. It is visible at distances of several hundred miles and is capable of producing injury to the eyes over large area (§ 12.79 et seq.).
2.60 Additional important effects that result from high-altitude bursts are the widespread ionization and other disturbances of the portion of the upper atmosphere known as the ionosphere. These disturbances affect the propagation of radio and radar waves, sometimes over extended areas (see Chapter X). Following the TEAK event, propagation of high-frequency (HF) radio communications (Table 10.91) was degraded over a region of several thousand miles in diameter for a period lasting from shortly after midnight until sunrise. Some very-high-frequency (VHF) communications circuits in the Pacific area were unable to function for about 30 seconds after the STARFISH PRIME event.
2.61 Detonations above about 19 miles can produce EMP effect (§ 2.46) on the ground over large areas, increasing with the yield of the explosion and the height of burst. For fairly large yields and burst heights, the EMP fields may be significant at nearly all points within the line of sight, i.e., to the horizon, from the burst point. Although these fields are weaker than those in the close-in region surrounding a surface burst, they are of sufficient magnitude to damage some unprotected electrical and electronic equipment. The mechanism of formation and the effects of the EMP are treated in Chapter XI.
2.62 An interesting visible effect of high-altitude nuclear explosions is the creation of an “ artificial aurora.” Within a second or two after burst time of the TEAK shot a brilliant aurora appeared from the bottom of the fireball and purple streamers were seen to spread toward the north. Less than a second later, an aurora was observed at Apia, in the Samoan Islands, more than 2,000 miles from the point of burst, although at no time was the fireball in direct view. The formation of the aurora is attributed to the motion along the lines of the earth’s magnetic field of beta particles (electrons), emitted by the radioactive fission fragments. Because of the natural cloud cover over Johnston Island at the time of burst, direct observation of the ORANGE fireball was not possible from the ground. However, such observations were made from aircraft flying above the low clouds. The auroras were less marked than from the TEAK shot, but an aurora lasting 17 minutes was again seen from Apia. Similar auroral effects were observed after the other high-altitude explosions mentioned in § 2.52.
DESCRIPTION OF UNDERWATER BURSTS
SHALLOW UNDERWATER EXPLOSION PHENOMENA
2.63 Certain characteristic phenomena are associated with an underwater nuclear explosion, but the details vary with the energy yield of the weapon, the distance below the surface at which the detonation occurs, and the depth and area of the body of water. The description given here is based mainly on the observations made at the BAKER test at Bikini in July 1946. In this test, a nuclear weapon of approximately 20-kilotons yield was detonated well below the surface of the lagoon which was about 200 feet deep. These conditions may be regarded as corresponding to a shallow underwater explosion.
2.64 In an underwater nuclear detonation, a fireball is formed, but it is smaller than for an air burst. At the BAKER test the water in the vicinity of the explosion was illuminated by the fireball. The distortion caused by the water waves on the surface of the lagoon prevented a clear view of the fireball, and the general effect was similar to that of light seen through a ground-glass screen. The luminosity persisted for a few thousandths of a second, but it disappeared as soon as the bubble of hot, high-pressure gases (or vapors) and steam constituting the fireball reached the water surface. At this time, the gases were expelled and cooled, so that the fireball was no longer visible.
2.65 In the course of its rapid expansion, the hot gas bubble, while still underwater, initiates a shock wave. Intersection of the shock wave with the surface produces an effect which, viewed from above, appears to be a rapidly expanding ring of darkened water. This is often called the “slick” because of its resemblance to an oil slick. Following closely behind the dark region is a white circular patch called the “crack,” probably caused by reflection of the water shock wave at the surface.
2.66 Immediately after the appearance of the crack, and prior to the formation of the Wilson cloud (§ 2.48), a mound or column of broken water and spray, called the “spray dome,” is thrown up over the point of burst (Fig. 2.66). This dome is caused by the velocity imparted to the water near the surface by the reflection of the shock wave and to the subsequent breakup of the surface layer into drops of spray. The initial upward velocity of the water is proportional to the pressure of the direct shock wave, and so it is greatest directly above the detonation point. Consequently, the water in the center rises more rapidly (and for a longer time) than water farther away. As a result, the sides of the spray dome become steeper as the water rises. The upward motion is terminated by the downward pull of gravity and the resistance of the air. The total time of rise and the maximum height depend upon the energy of the explosion, and upon its depth below the water surface. Additional slick, crack, and spray-dome phenomena may result if the shock wave reflected from the water bottom and compression waves produced by the gas bubble (§ 2.86 et seq.) reach the surface with sufficient intensity.
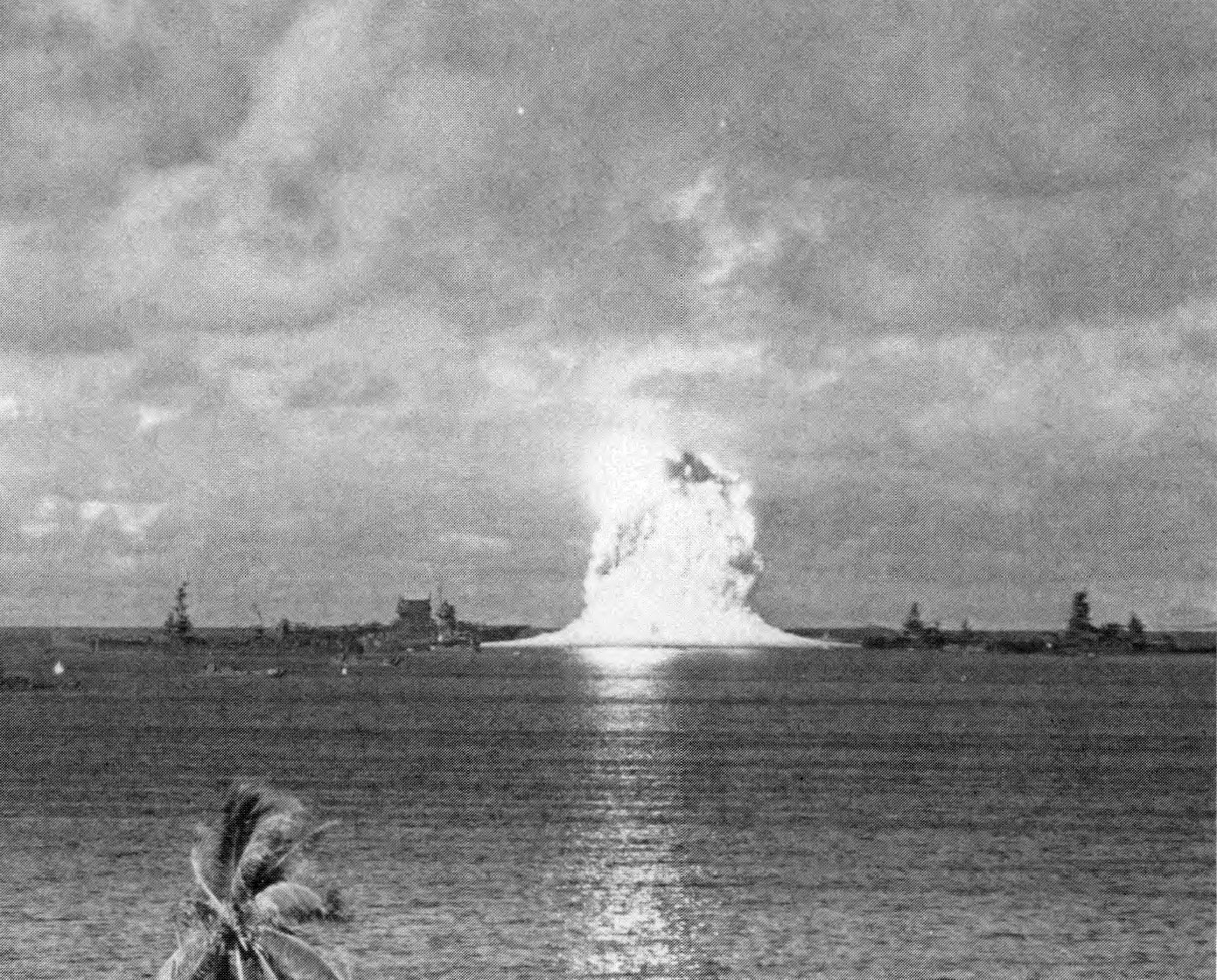
2.67 If the depth of burst is not too great, the bubble remains essentially intact until it rises to the surface of the water. At this point the steam, fission gases, and debris are expelled into the atmosphere. Part of the shock wave passes through the surface into the air, and because of the high humidity the conditions are suitable for the formation of a condensation cloud (Fig. 2.67a). As the pressure of the bubble is released, water rushes into the cavity, and the resultant complex phenomena cause the water to be thrown up as a hollow cylinder or chimney of spray called the “column” or “plume.” The radioactive contents of the bubble are vented through this hollow column and may form a cauliflower-shaped cloud at the top (Fig. 2.67b.)
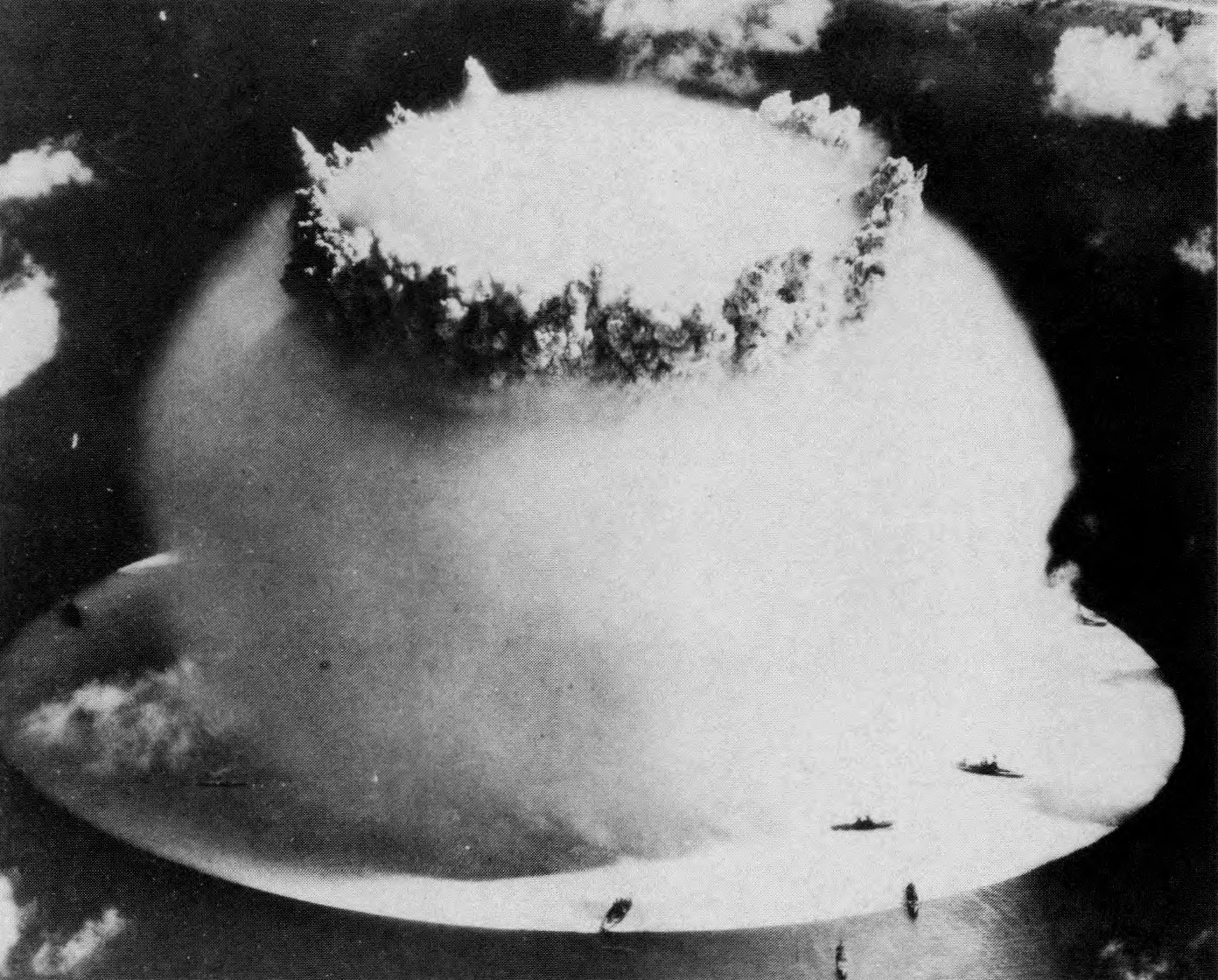
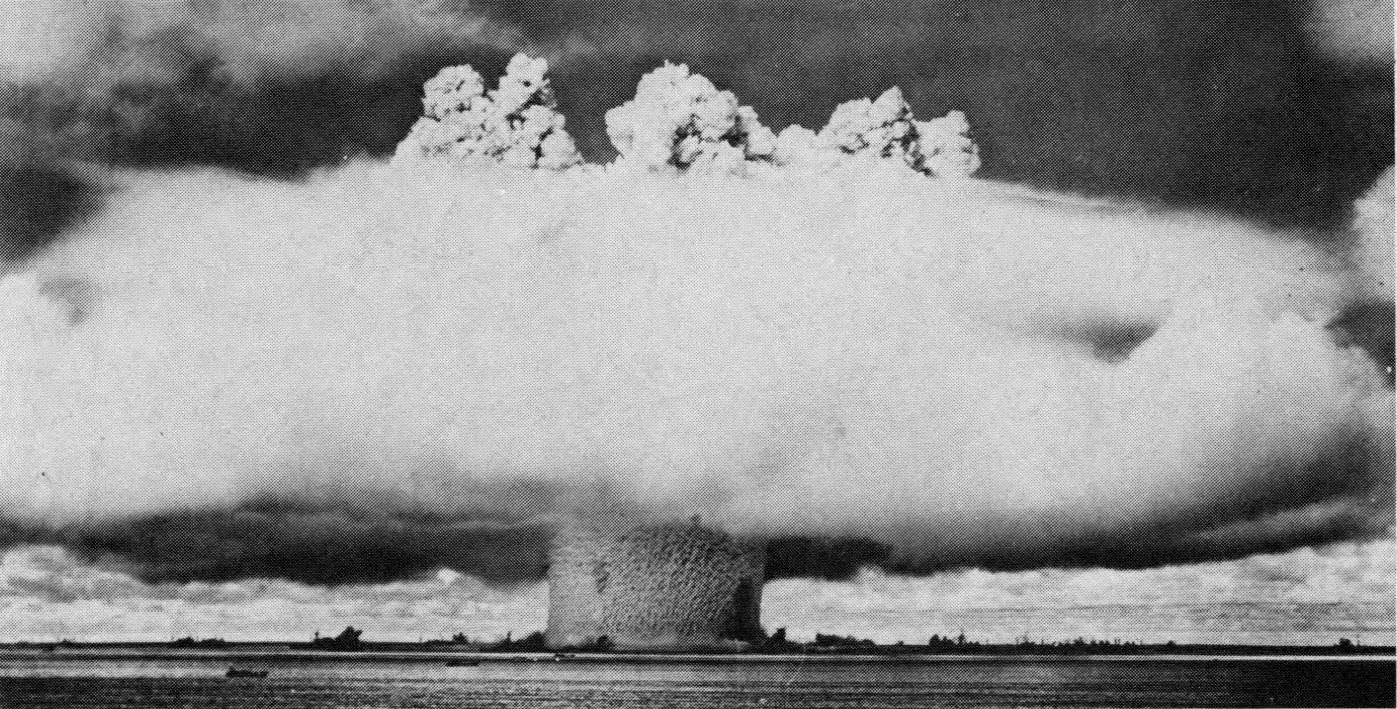
2.68 In the shallow underwater (BAKER) burst at Bikini, the spray dome began to form at about 4 milliseconds after the explosion. Its initial rate of rise was roughly 2,500 feet per second, but this was rapidly diminished by air resistance and gravity. A few milliseconds later, the hot gas bubble reached the surface of the lagoon and the column began to form, quickly overtaking the spray dome. The maximum height attained by the hollow column, through which the gases vented, could not be estimated exactly because the upper part was surrounded by the radioactive cloud (Fig. 2.68). The column was probably some 6,000 feet high and the maximum diameter was about 2,000 feet. The walls were probably 300 feet thick, and approximately a million tons of water were raised in the column.
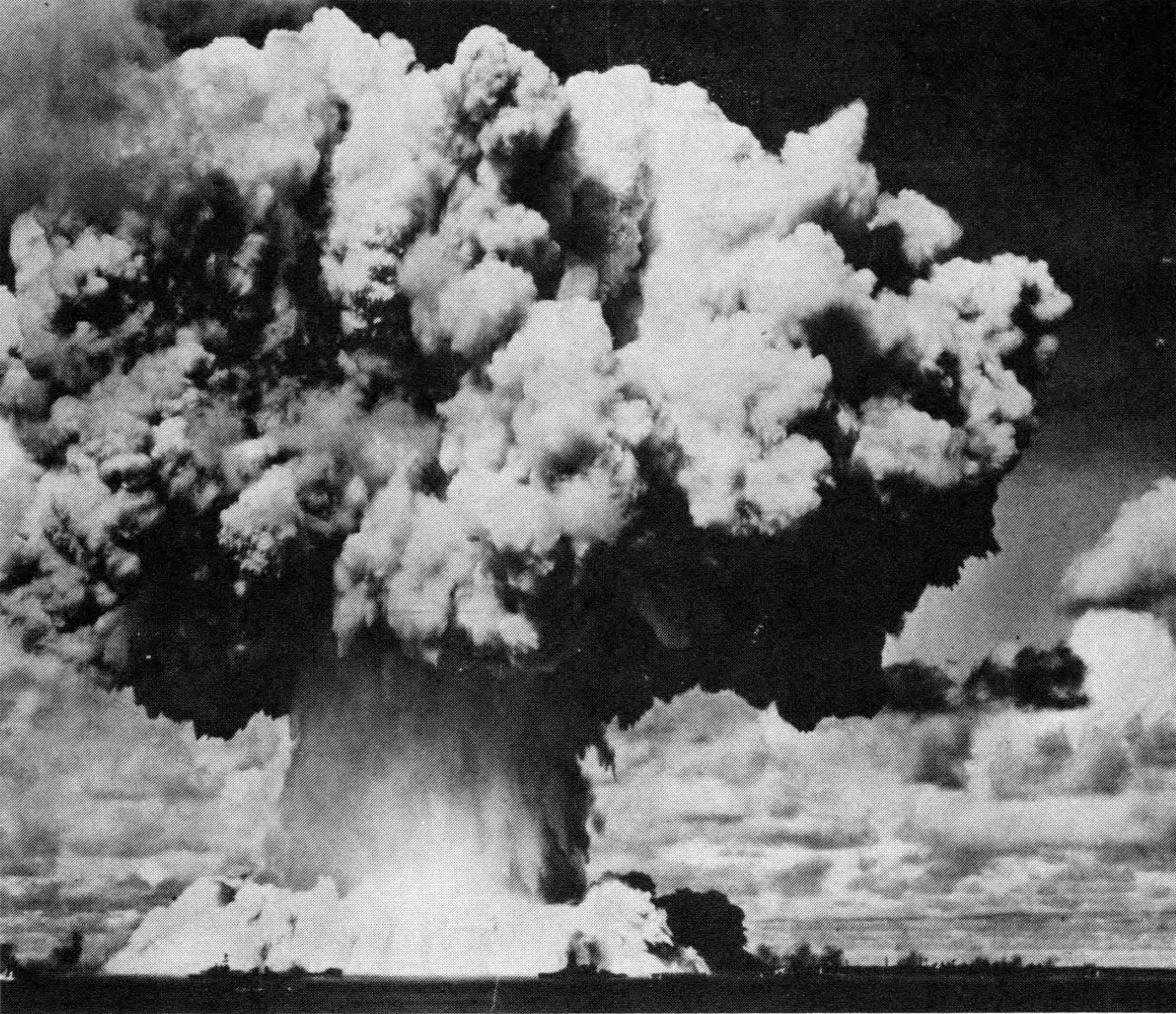
2.69 The cauliflower-shaped cloud, which concealed part of the upper portion of the column, contained some of the fission products and other weapon residues, as well as a large quantity of water in small droplet form. In addition, there is evidence that material sucked up from the bottom of the lagoon was also present, for a calcareous (or chalky) sediment, which must have dropped from this cloud, was found on the decks of ships some distance from the burst. The cloud was roughly 6,000 feet across and ultimately rose to a height of nearly 10,000 feet before being dispersed. This is considerably less than the height attained by the radioactive cloud in an air burst.
2.70 The disturbance created by the underwater burst caused a series of waves to move outward from the center of the explosion across the surface of Bikini lagoon. At 11 seconds after the detonation, the first wave had a maximum height of 94 feet and was about 1,000 feet from surface zero. This moved outward at high speed and was followed by a series of other waves. At 22,000 feet from surface zero, the ninth wave in the series was the highest with a height of 6 feet.
2.71 It has been observed that certain underwater and water surface bursts have caused unexpectedly serious flooding of nearby beach areas, the depth of inundation being sometimes twice as high as the approaching water wave. The extent of inundation is related in a complex manner to a number of factors which include the energy yield of the explosion, the depth of burst, the depth of the water, the composition and contour of the bottom, and the angle the approaching wave makes with the shoreline.
THE VISIBLE BASE SURGE
2.72 As the column (or plume) of water and spray fell back into the lagoon in the BAKER test, there developed a gigantic wave (or cloud) of mist completely surrounding the column at its base (Fig. 2.68). This doughnut-shaped cloud, moving rapidly outward from the column, is called the “base surge.” It is essentially a dense cloud of small water droplets, much like the spray at the base of Niagara Falls (or other high waterfalls), but having the property of flowing almost as if it were a homogeneous fluid.
2.73 The base surge at Bikini commenced to form at l0 or 12 seconds after the detonation. The surge cloud, billowing upward, rapidly attained a height of 900 feet, and moved outward at an initial rate of more than a mile a minute. Within 4 minutes the outer radius of the cloud, growing rapidly at first and then more slowly, was nearly 3½ miles across and its height had then increased to 1,800 feet. At this stage, the base surge gradually rose from the surface of the water and began to merge with the radioactive cloud and other clouds in the sky (Fig. 2.73).
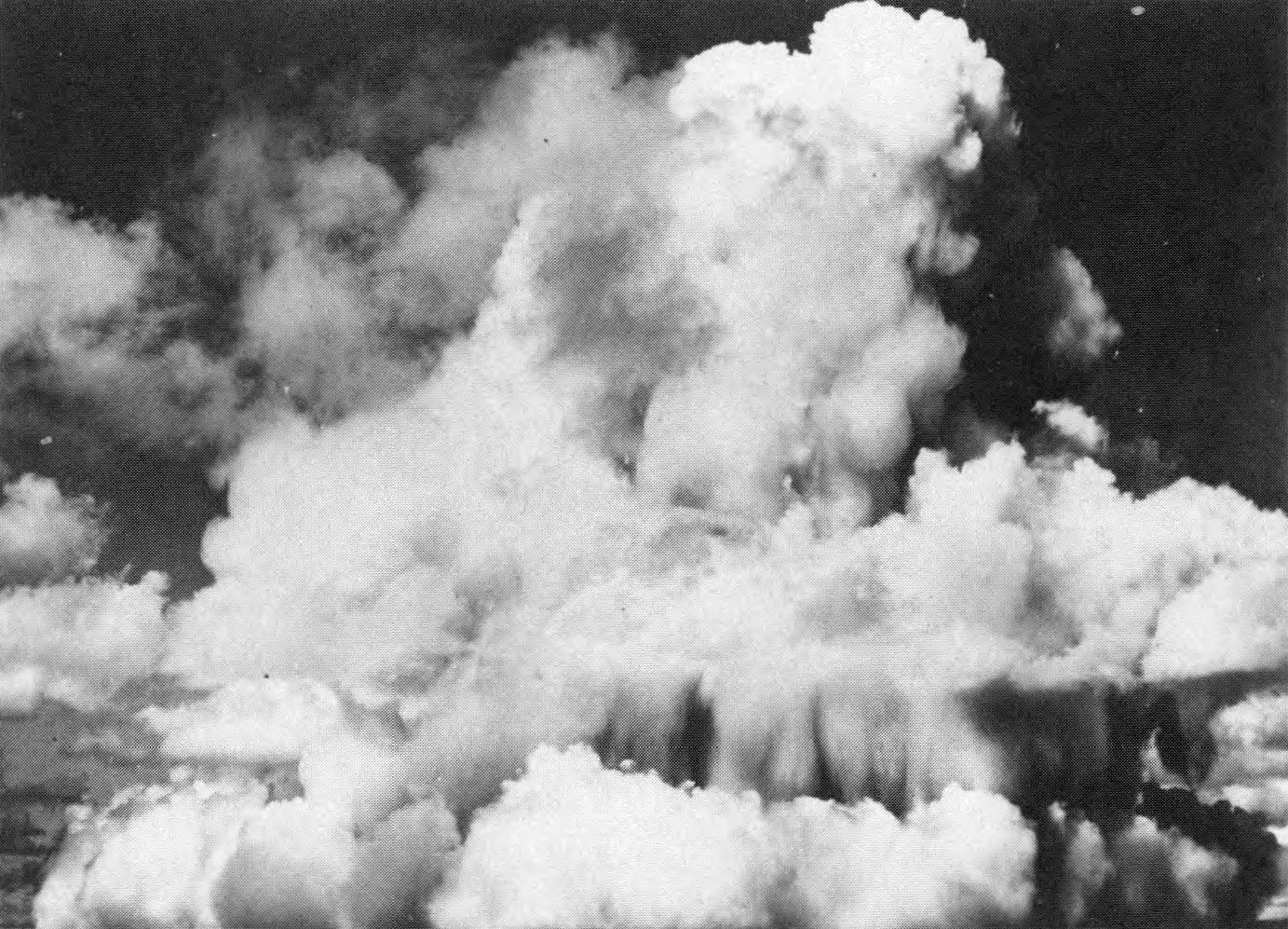
2.74 After about 5 minutes, the base surge had the appearance of a mass of stratocumulus clouds which eventually reached a thickness of several thousand feet (Fig. 2.74). A moderate to heavy rainfall, moving with the wind and lasting for nearly an hour, developed from the cloud mass. In its early stages the rain was augmented by the small water droplets still descending from the radioactive cloud.
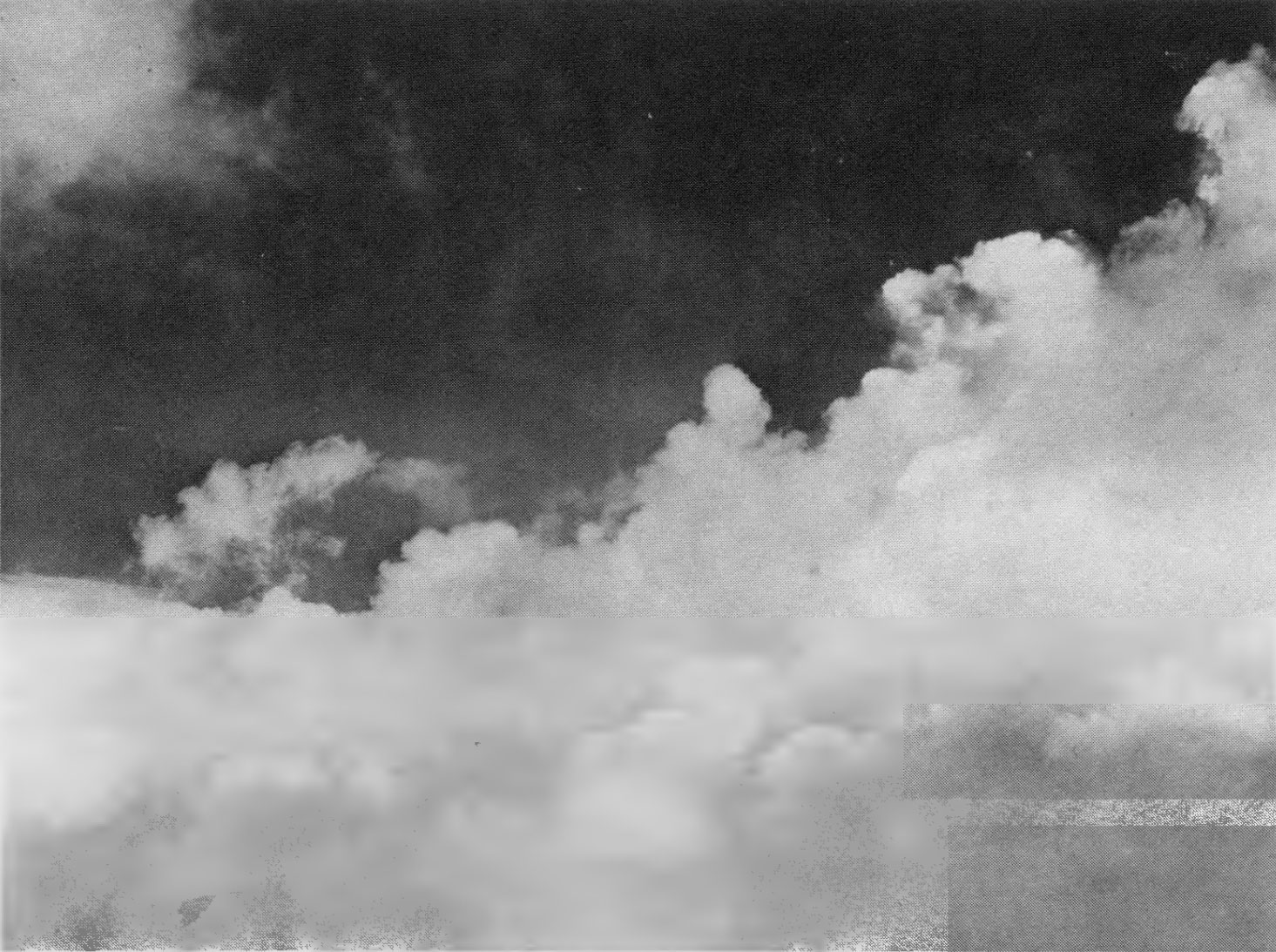
2.75 In the few instances in which base surge formation has been observed over water, the visible configuration has been quite irregular. Nevertheless, to a good approximation, the base surge can be represented as a hollow cylinder with the inner diameter about two-thirds of the outer diameter. The heights of the visible base surge clouds have generally ranged between 1,000 and 2,000 feet.
2.76 The necessary conditions for the formation of a base surge have not been definitely established, although it is reasonably certain that no base surge would accompany bursts at great depths. The underwater test shots upon which the present analysis is based have all created both a visible and an invisible (§ 2.77) base surge. The only marked difference between the phenomena at the various tests is that at Bikini BAKER there was an airborne cloud, evidently composed of fission debris and steam. The other shots, which were at somewhat greater depths, produced no such cloud. The whole of the plume fell back into the surface of the water where the low-lying base surge cloud was formed.
THE RADIOACTIVE BASE SURGE
2.77 From the weapons effects standpoint, the importance of the base surge lies in the fact that it is likely to be highly radioactive because of the fission (and other) residues present either at its inception, or dropped into it from the radioactive cloud. Because of its radioactivity, it may represent a hazard for a distance of several miles, especially in the downwind direction. The fission debris is suspended in the form of very small particles that occupy the same volume as the visible base surge at early times, that is, within the first 3 or 4 minutes. However, when the small water droplets which make the base surge visible evaporate and disappear, the radioactive particles and gases remain in the air and continue to move outwards as an invisible radioactive base surge. There may well be some fallout or rainout on to the surface of the water (or ship or shore station) from the radioactive base surge, but in many cases it is expected to pass over without depositing any debris. Thus, according to circumstances, there may or may not be radioactive contamination on the surfaces of objects in the vicinity of a shallow underwater nuclear burst.
2.78 The radioactive base surge continues to expand in the same manner as would have been expected had it remained visible. It drifts downwind either as an invisible, doughnut-shaped cloud or as several such possibly concentric clouds that approximate a lowlying disc with no hole in the center. The latter shape is more probable for deeper bursts. The length of time this base surge remains radioactive will depend on the energy yield of the explosion, the burst depth, and the nearness of the sea bottom to the point of burst. In addition, weather conditions will control depletion of debris due to rainout and diffusion by atmospheric winds. As a general rule, it is expected that there will be a considerable hazard from the radioactive base surge within the first 5 to 10 minutes after an underwater explosion and a decreasing hazard for half an hour or more.
2.79 The proportion of the residual nuclear radiation that remains in the water or that is trapped by the falling plume and returns immediately to the surface is determined by the location of the burst and the depth of the water, and perhaps also by the nature of the bottom material. Although as much as 90 percent of the fission product and other radioactivity could be left behind in the water, the base surge, both visible and invisible, could still be extremely radioactive in its early stages.
THERMAL AND NUCLEAR RADIATIONS IN UNDERWATER BURST
2.80 Essentially all the thermal radiation emitted by the fireball while it is still submerged is absorbed by the surrounding water. When the hot steam and gases reach the surface and expand, the cooling is so rapid that the temperature drops almost immediately to a point where there is no further appreciable emission of thermal radiation. It follows, therefore, that in an underwater nuclear explosion the thermal radiation can be ignored, as far as its effects on people and as a source of fire are concerned.
2.81 It is probable, too, that most of the neutrons and gamma rays liberated within a short time of the initiation of the explosion will also be absorbed by the water. But, when the fireball reaches the surface and vents, the gamma rays (and beta particles) from the fission products will represent a form of initial nuclear radiation. In addition, the radiation from the radioactive residues present in the column, cloud, and base surge, all three of which are formed within a few seconds of the burst, will contribute to the initial effects.
2.82 However, the water fallout (or rainout) from the cloud and the base surge are also responsible for the residual nuclear radiation, as described above. For an underwater burst, it is thus less meaningful to make a sharp distinction between initial and residual radiations, such as is done in the case of an air burst. The initial nuclear radiations merge continuously into those which are produced over a period of time following the nuclear explosion.
DEEP UNDERWATER EXPLOSION PHENOMENA
2.83 Because the effects of a deep underwater nuclear explosion are largely of military interest, the phenomena will be described in general terms and in less detail than for a shallow underwater burst. The following discussion is based largely on observations made at the WAHOO shot in 1958, when a nuclear weapon was detonated at a depth of 500 feet in deep water. The generation of large-scale water waves in deep underwater bursts will be considered in Chapter VI.
2.84 The spray dome formed by the WAHOO explosion rose to a height of 900 feet above the surface of the water (Fig. 2.84a). Shortly after the maximum height was attained, the hot gas and steam bubble burst through the dome, throwing out a plume with jets in all directions; the highest jets reached an elevation of 1,700 feet (Fig. 2.84b). There was no airborne radioactive cloud, such as was observed in the shallow underwater BAKER shot. The collapse of the plume created a visible base surge extending out to a distance of over 2 ½ miles downwind and reaching a maximum height of about 1,000 feet (Fig. 2.84c). This base surge traveled outward at an initial speed of nearly 75 miles per hour, but decreased within 10 seconds to less than 20 miles per hour.
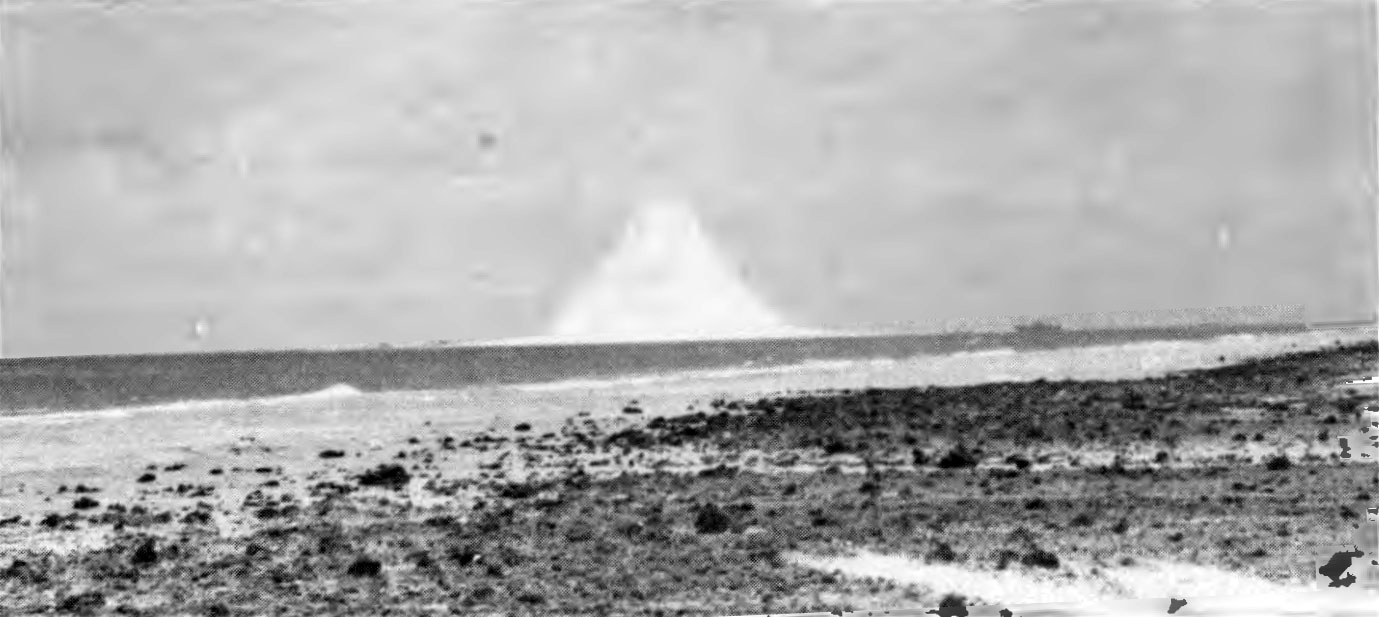
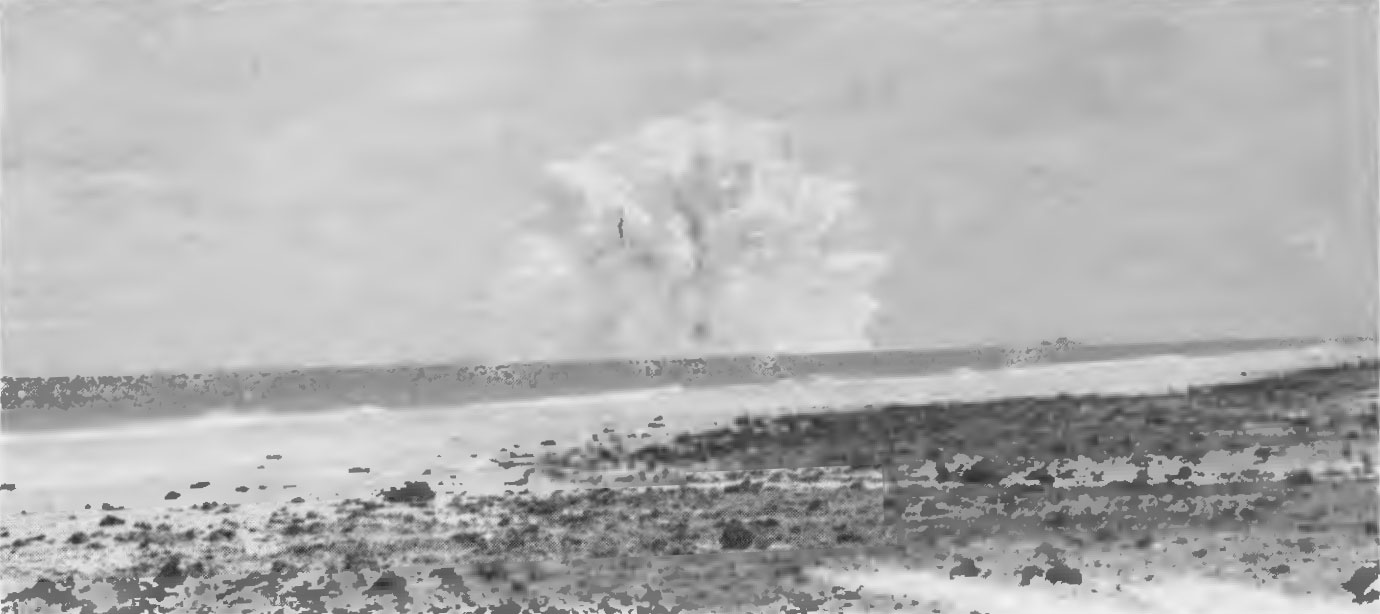
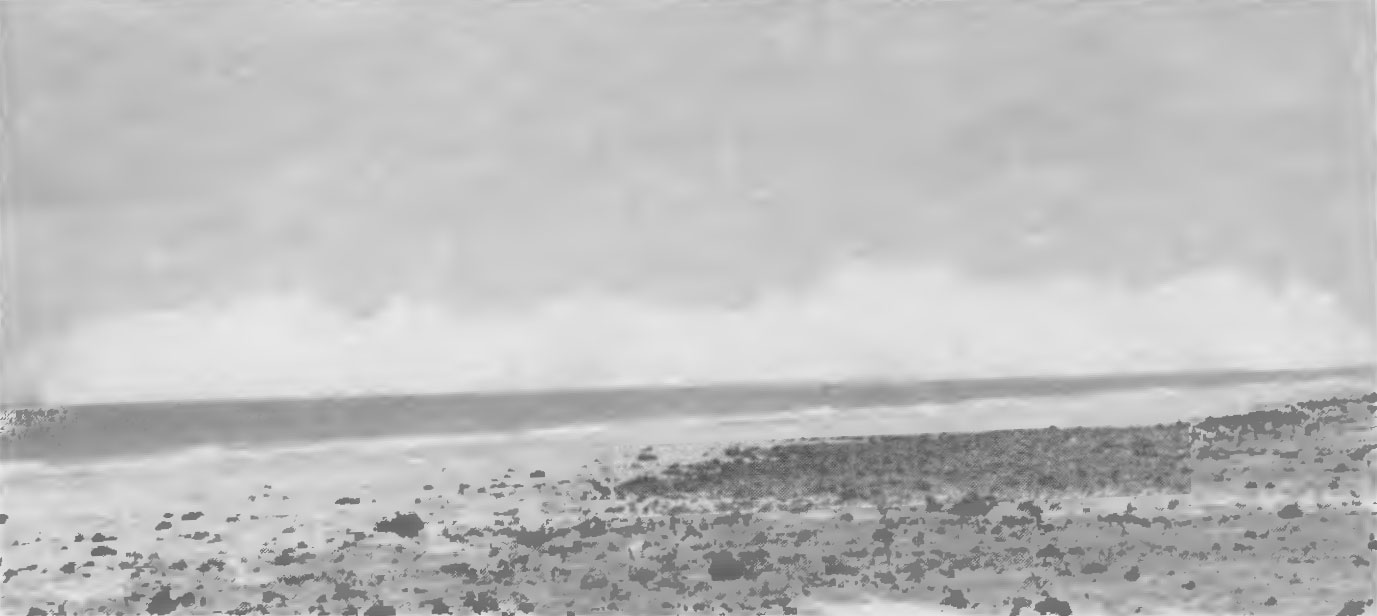
2.85 There was little evidence of the fireball in the WAHOO shot, because of the depth of the burst, and only a small amount of thermal radiation escaped. The initial nuclear radiation was similar to that from a shallow underwater burst, but there was no lingering airborne radioactive cloud from which fallout could occur. The radioactivity was associated with the base surge while it was visible and also after the water droplets had evaporated. The invisible, radioactive base surge continued to expand while moving in the downwind direction. However, very little radioactivity was found on the surface of the water.
2.86 The hot gas bubble formed by a deep underwater nuclear explosion rises through the water and continues to expand at a decreasing rate until a maximum size is reached. If it is not too near the surface or the bottom at this time, the bubble remains nearly spherical. As a result of the outward momentum of the water surrounding the bubble, the latter actually overexpands; that is to say, when it attains its maximum size its contents are at a pressure well below the ambient water pressure. The higher pressure outside the bubble then causes it to contract, resulting in an increase of the pressure within the bubble and condensation of some of the steam. Since the hydrostatic (water) pressure is larger at the bottom of the bubble than at the top, the bubble does not remain spherical during the contraction phase. The bottom moves upward faster than the top (which may even remain stationary) and reaches the top to form a toroidal bubble as viewed from above. This causes turbulence and mixing of the bubble contents with the surrounding water.
2.87 The momentum of the water set in motion by contraction of the bubble causes it to overcontract, and its internal pressure once more becomes higher than the ambient water pressure. A second compression (shock) wave in the water commences after the bubble reaches its minimum volume. This compression wave has a lower peak overpressure but a longer duration than the initial shock wave in the water. A second cycle of bubble expansion and contraction then begins.
2.88 If the detonation occurs far enough below the surface, as in the WIGWAM test in 1955 at a depth of about 2,000 feet, the bubble continues to pulsate and rise, although after three complete cycles enough steam will have condensed to make additional pulsations unlikely. During the pulsation and upward motion of the bubble, the water surrounding the bubble acquires considerable upward momentum and eventually breaks through the surface with a high velocity, e.g., 200 miles per hour in the WIGWAM event, thereby creating a large plume. If water surface breakthrough occurs while the bubble pressure is below ambient, a phenomenon called “blowin” occurs. The plume is then likely to resemble a vertical column which may break up into jets that disintegrate into spray as they travel through the air.
2.89 The activity levels of the radioactive base surge will be affected by the phase of the bubble when it breaks through the water surface. Hence, these levels may be expected to vary widely, and although the initial radiation intensities may be very high, their duration is expected to be short.
DESCRIPTION OF UNDERGROUND BURSTS
SHALLOW UNDERGROUND EXPLOSION PHENOMENA
2.90 For the present purpose, a shallow underground explosion may be regarded as one which produces a substantial crater resulting from the throwout of earth and rock. There is an optimum depth of burst, dependent on the energy yield of the detonation and the nature of the rock medium, which gives a crater of maximum size. The mechanism of the formation of such throwout (or excavation) craters will be considered here. For shallower depths of burst, the behavior approaches that of a surface burst (§§ 2.18, 6.03 et seq.), whereas for explosions at greater depths the phenomena tend toward those of a deep underground detonation (§ 2. 101 et seq.).
2.91 When a nuclear weapon is exploded under the ground, a sphere of extremely hot, high-pressure gases, including vaporized weapon residues and rock, is formed. This is the equivalent of the fireball in an air or surface burst. The rapid expansion of the gas bubble initiates a ground shock wave which travels in all directions away from the burst point. When the upwardly directed shock (compression) wave reaches the earth’s surface, it is reflected back as a rarefaction (or tension) wave. If the tension exceeds the tensile strength of the surface material, the upper layers of the ground will spall, i.e., split off into more-or-less horizontal layers. Then, as a result of the momentum imparted by the incident shock wave, these layers move upward at a speed which may be about 150 (or more) feet per second.
2.92 When it is reflected back from the surface, the rarefaction wave travels into the ground toward the expanding gas sphere (or cavity) produced by the explosion. If the detonation is not at too great a depth, this wave may reach the top of the cavity while it is still growing. The resistance of the ground to the upward growth of the cavity is thus decreased and the cavity expands rapidly in the upward direction. The expanding gases and vapors can thus supply additional energy to the spalled layers, so that their upward motion is sustained for a time or even increased. This effect is referred to as “gas acceleration.”
2.93 The ground surface moving upward first assumes the shape of a dome. As the dome continues to increase in height, cracks form through which the cavity gases vent to the atmosphere. The mound then disintegrates completely and the rock fragments are thrown upward and outward (Fig. 2.93). Subsequently, much of the ejected material collapses and falls . back, partly into the newly formed crater and partly onto the surrounding “lip.” The material that falls back immediately into the crater is called the “fallback,” whereas that descending on the lip is called the “ejecta.” The size of the remaining (or “apparent”) crater depends on the energy yield of the detonation and on the nature of the excavated medium. In general, for equivalent conditions, the volume of the crater is roughly proportional to the yield of the explosion.
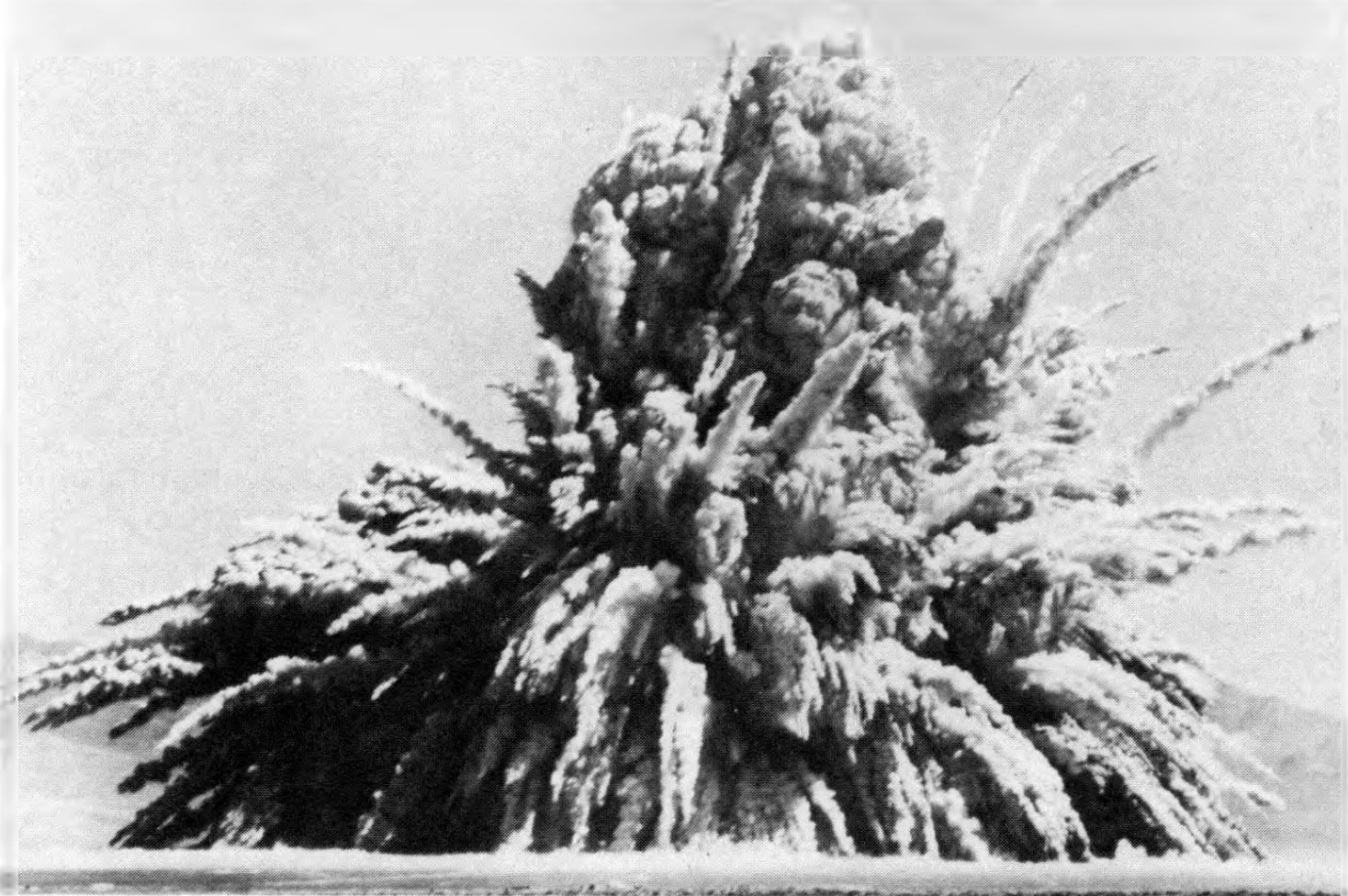
2.94 The relative extents to which spalling and gas acceleration contribute to the formation of a throwout crater depend to large extent on the moisture content of the rock medium. In rock containing a moderately large proportion of water, the cavity pressure is greatly increased by the presence of water vapor. Gas acceleration then plays an important role in crater formation. In dry rock, however, the contribution of gas acceleration to the upward motion of the ground is generally small and may be unobservable.
2.95 As in an underwater burst, part of the energy released by the weapon in a shallow underground explosion appears as an air blast wave. The fraction of the energy imparted to the air in the form of blast depends primarily on the depth of burst for the given total energy yield. The greater the depth of burst, the smaller, in general, will be the proportion of shock energy that escapes into the air. For a sufficiently deep explosion, there is, of course, no blast wave.
BASE SURGE AND MAIN CLOUD
2.96 When the tailback from a shallow underground detonation descends to the ground, it entrains air and fine dust particles which are carried downward. The dust-laden air upon reaching the ground moves outward as a result of its momentum and density, thereby producing a base surge, similar to that observed in shallow underwater explosions. The base surge of dirt particles moves outward from the center of the explosion and is subsequently carried downwind. Eventually the particles settle out and produce radioactive contamination over a large area, the extent of which depends upon the depth of burst, the nature of the soil, and the atmospheric conditions, as well as upon the energy yield of the explosion. A dry sandy terrain would be particularly conducive to base surge formation in an underground burst.
2.97 Throwout crater formation is apparently always accompanied by a base surge. If gas acceleration occurs, however, a cloud consisting of particles of various sizes and the hot gases escaping from the explosion cavity generally also forms and rises to a height of thousands of feet. This is usually referred to as the “main cloud,” to distinguish it from the base surge cloud. The latter surrounds the base of the main cloud and spreads out initially to a greater distance. The main cloud and base surge formed in the SEDAN test (100 kilotons yield, depth of burial 635 feet in alluvium containing 7 percent of water) are shown in the photograph in Fig. 2. 97, taken six minutes after the explosion.
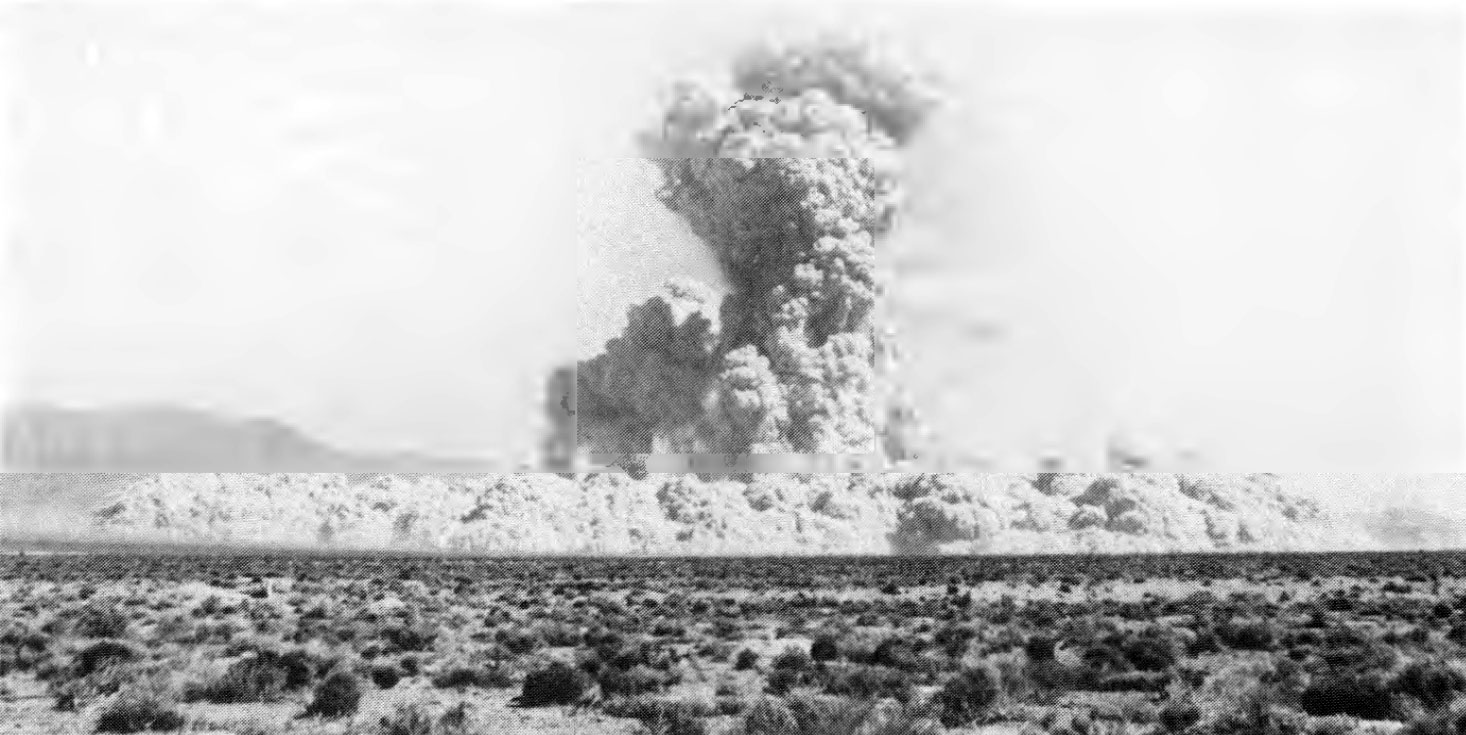
2.98 Both the base surge and the main cloud are contaminated with radioactivity, and the particles present contribute to the fallout. The larger pieces are the first to reach the earth and so they are deposited near the location of the burst. But the smaller particles remain suspended in the air some time and may be carried great distances by the wind before they eventually settle out.
THERMAL AND NUCLEAR RADIATIONS IN UNDERGROUND BURSTS
2.99 The situations as regards thermal and nuclear radiations from an underground burst are quite similar to those described above in connection with an underwater explosion. As a general rule, the thermal radiation is almost completely absorbed by the ground material, so that it does not represent a significant hazard. Most of the neutrons and early gamma rays are also removed, although the capture of the neutrons may cause a considerable amount of induced radioactivity in various materials present in the soil (§ 9.35). This will constitute a small part of the residual nuclear radiation, of importance only in the close vicinity of the point of burst. The remainder of the residual radiation will be due to the contaminated base surge and fallout.
2.100 For the reasons given in § 2.82 for an underwater burst, the initial and residual radiations from an underground burst tend to merge into one another. The distinction which is made in the case of air and surface bursts is consequently less significant in a subsurface explosion.
DEEP UNDERGROUND EXPLOSION PHENOMENA
2.101 A deep underground explosion is one occurring at such a depth that the effects are essentially fully contained. The surface above the detonation point may be disturbed, e.g., by the formation of a shallow subsidence crater or a mound, and ground tremors may be detected at a distance. There is no significant venting of the weapon residues to the atmosphere, although some of the noncondensable gases present may seep out gradually through the surface. The United States has conducted many deep underground tests, especially since September 1961. Almost all of the explosion energy has been contained in the ground, and, except in the few cases of accidental venting or seepage of a small fraction of the residues, the radioactivity from these explosions has also been confined. The phenomena of deep underground detonations can be described best in terms of four phases having markedly different time scales.
2.102 First, the explosion energy is released in less than one-millionth part of a second, i.e., less than one microsecond (§ 1.54 footnote). As a result, the pressure in the hot gas bubble formed will rise to several million atmospheres and the temperature will reach about a million degrees within a few microseconds. In the second (hydrodynamic) stage, which generally is of a few tenths of a second duration, the high pressure of the hot gases initiates a strong shock wave which breaks away and expands in all directions with a velocity equal to or greater than the speed of sound in the rock medium. During the hydrodynamic phase, the hot gases continue to expand, although more slowly than initially, and form a cavity of substantial size. At the end of this phase the cavity will have attained its maximum diameter and its walls will be lined with molten rock. The shock wave will have reached a distance of some hundreds of feet ahead of the cavity and it will have crushed or fractured much of the rock in the region it has traversed. The shock wave will continue to expand and decrease in strength eventually becoming the “head” (or leading) wave of a train of seismic wave (§ 6.19). During the third stage, the cavity will cool and the molten rock material will collect and solidify at the bottom of the cavity.
2.103 Finally, the gas pressure in the cavity decreases to the point when it can no longer support the overburden. Then, in a matter of seconds to hours, the roof falls in and this is followed by progressive collapse of the overlying rocks. A tall cylinder, commonly referred to as a “chimney,” filled with broken rock or rubble is thus formed (Fig. 2.103). If the top of the chimney does not reach the ground surface, an empty space, roughly equivalent to the cavity volume, will remain at the top of the chimney. However, if the collapse of the chimney material should reach the surface, the ground will sink into to the empty space thereby forming a subsidence crater (see Fig. 6.06f). The collapse of the roof and the formation of the chimney represented the fourth (and last) phase of the underground explosion.
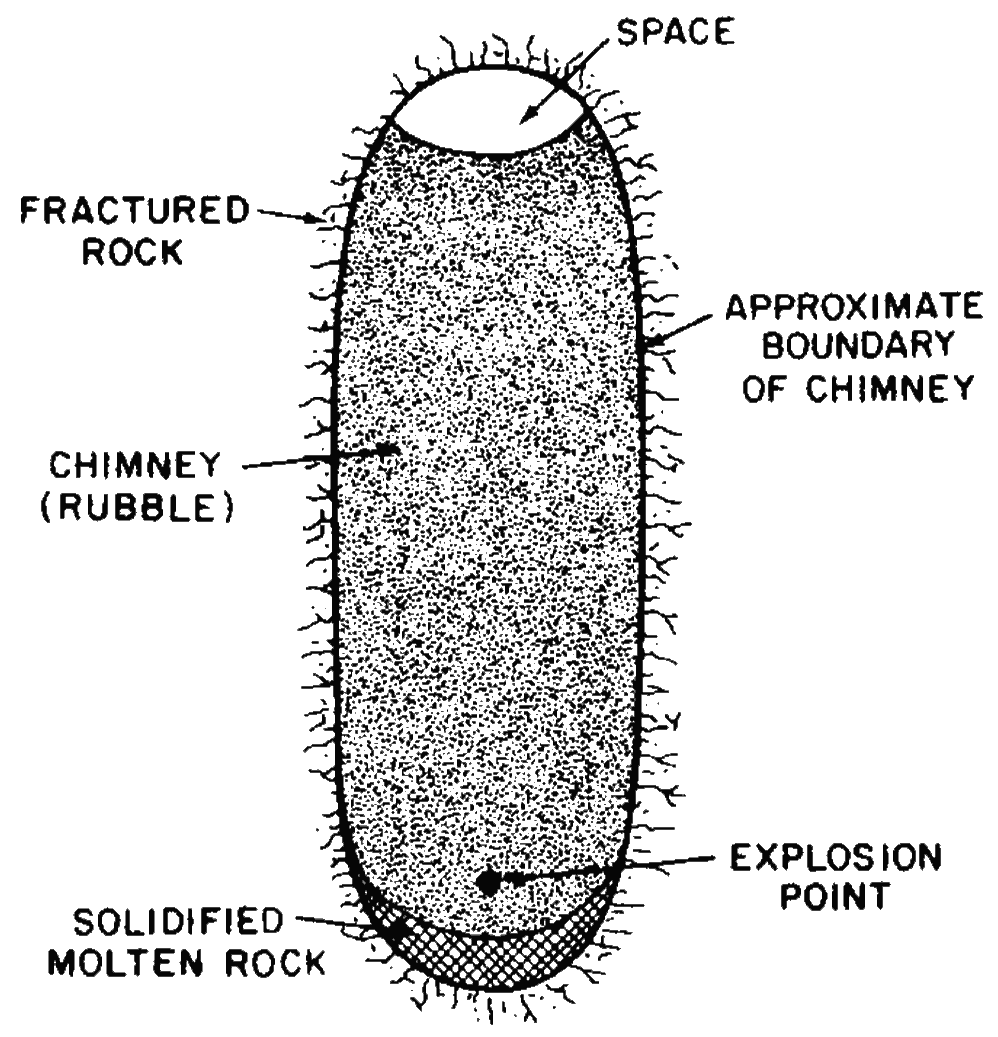
2.104 The effects of the RAINIER event of Operation Plumbbob in 1957 will provide an example of the extent to which the surrounding medium may be affected by a deep underground detonation. RAINIER was a 1.7-kiloton nuclear device detonated in a chamber 6 x 6 x 7 feet in size, at a depth of 790 feet below the surface in a compacted volcanic-ash medium referred to geologically as “tuff. “ During the hydrodynamic stage the chamber expanded to form a spherical cavity 62 feet in radius, which was lined with molten rock about 4 inches thick. The shock from the explosion crushed the surrounding medium to a radius of 130 feet and fractured it to 180 feet. Seismic signals were detected out to distances of several hundred miles and a weak signal was recorded in Alaska. The chimney extended upward for about 400 feet from the burst point. Further information on cavity and chimney dimensions is given in Chapter VI.
2.105 Deep underground nuclear detonations, especially those of high yield, are followed by a number of minor seismic tremors called “aftershocks,” the term that is used to describe the secondary tremors that generally occur after the main shock of a large earthquake. In tests made in Nevada and on Amchitka Island in the Aleutians, the aftershocks have not constituted a danger to people or to structures off the test sites. No correlation has been found between underground nuclear detonations and the occurrence of natural earthquakes in the vicinity (§ 6.24 et seq.).
SCIENTIFIC ASPECTS OF NUCLEAR EXPLOSION PHENOMENA7
INTRODUCTION
2.106 The events which follow the very large and extremely rapid energy release in a nuclear explosion are mainly the consequences of the interaction of the kinetic energy of the fission fragments and the thermal radiations with the medium surrounding the explosion. The exact nature of these interactions, and hence the directly observable and indirect effects they produce, that is to say, the nuclear explosion phenomena, are dependent on such properties of the medium as its temperature, pressure, density, and composition. It is the variations in these factors in the environment of the nuclear detonation that account for the different types of response associated with air, high-altitude, surface, and subsurface bursts, as described earlier in this chapter.
2.107 Immediately after the explosion time, the temperature of the weapon material is several tens of million degrees and the pressures are estimated to be many million atmospheres. As a result of numerous inelastic collisions, part of the kinetic energy of the fission fragments is converted into internal and radiation energy. Some of the electrons are removed entirely from the atoms, thus causing ionization, whereas others are raised to higher energy (or excited) states while still remaining attached to the nuclei. Within an extremely short time, perhaps a hundredth of a microsecond or so, the weapon residues consist essentially of completely and partially stripped (ionized) atoms, many of the latter being in excited states, together with the corresponding free electrons. The system then immediately emits electromagnetic (thermal) radiation, the nature of which is determined by the temperature. Since this is of the order of several times 107 degrees, most of the energy emitted within a microsecond or so is in the soft X-ray region (§ 1.77, see also § 7.75).
2.108 The primary thermal radiation leaving the exploding weapon is absorbed by the atoms and molecules of the surrounding medium. The medium is thus heated and the resulting fireball re-radiates part of its energy as the secondary thermal radiation of longer wavelengths (§ 2.38). The remainder of the energy contributes to the shock wave formed in the surrounding medium. Ultimately, essentially all the thermal radiation (and shock wave energy) is absorbed and appears as heat, although it may be spread over a large volume. In a dense medium such as earth or water, the degradation and absorption occur within a short distance from the explosion, but in air both the shock wave and the thermal radiation may travel considerable distances. The actual behavior depends on the air density, as will be seen later.
2.109 It is apparent that the kinetic energy of the fission fragments, constituting some 85 percent of the total energy released, will distribute itself between thermal radiation, on the one hand, and shock and blast, on the other hand, in proportions determined largely by the nature of the ambient medium. The higher the density of the latter, the greater the extent of the coupling between it and the energy from the exploding nuclear weapon. Consequently, when a burst takes place in a medium of high density, e.g., water or earth, a larger percentage of the kinetic energy of the fission fragments is converted into shock and blast energy than is the case in a less dense medium, e.g., air. At very high altitudes, on the other hand, where the air pressure is extremely low, there is no true fireball and the kinetic energy of the fission fragments is dissipated over a very large volume. In any event, the form and amount in which the thermal radiation is received at a distance from the explosion will depend on the nature of the intervening medium.
DEVELOPMENT OF THE FIREBALL IN AN AIR BURST
2.110 As seen above, most of the initial (or primary) thermal radiation from a nuclear explosion is in the soft X-ray region of the spectrum. If the burst occurs in the lower part of the atmosphere where the air density is appreciable, the X rays are absorbed in the immediate vicinity of the burst, and they heat the air to high temperatures. This sphere of hot air is sometimes referred to as the “X-ray fireball.” The volume of air involved; resultant air temperatures, and ensuing behavior of this fireball are all determined by the burst conditions. At moderate and low altitudes (below about 100,000 feet), the X rays are absorbed within some yards of the burst point, and the relatively small volume of air involved is heated to a very high temperature.
2.111 The energies (or wavelengths) of the X rays, as determined by the temperature of the weapon debris, cover a wide range (§ 7.73 et seq.), and a small proportion of the photons (§ 1.74) have energies considerably in excess of the average. These high-energy photons are not easily absorbed and so they move ahead of the fireball front. As a result of interaction with the atmospheric molecules, the X rays so alter the chemistry and radiation absorption properties of the air that, in the air burst at low and moderate altitudes, a veil of opaque air is generated that obscures the early growth of the fireball. Several microseconds elapse before the fireball front emerges from the opaque X-ray veil.
2.112 The X-ray fireball grows in size as a result of the transfer of radiation from the very hot interior where the explosion has occurred to the cooler exterior. During this “radiative growth” phase, most of the energy transfer in the hot gas takes place in the following manner. First, an atom, molecule, ion, or electron absorbs a photon of radiation and is thereby converted into an excited state. The atom or other particle remains in this state for a short time and then emits a photon, usually of lower energy. The residual energy is retained by the particle either as kinetic energy or as internal energy. The emitted photon moves off in a random direction with the velocity of light, and it may then be absorbed once again to form another excited particle. The latter will then re-emit a photon, and so on. The radiation energy is thus transmitted from one point to another within the gas; at the same time, the average photon energy (and radiation frequency) decreases. The energy lost by the photons serves largely to heat the gas through which the photons travel.
2.113 If the mean free path of the radiation, i.e., the average distance a photon travels between interactions, is large in comparison with the dimensions of the gaseous volume, the transfer of energy from the hot interior to the cooler exterior of the fireball will occur more rapidly than if the mean free path is short. This is because, in their outward motion through the gas, the photons with short mean free paths will be absorbed and re-emitted several times. At each re-emission the photon moves away in a random direction, and so the effective rate of transfer of energy in the outward direction will be less than for a photon of long mean free path which undergoes little or no absorption and re-emission in the hot gas.
2.114 In the radiative growth phase, the photon mean free paths in the hot fireball are of the order of (or longer than) the fireball diameter because at the very high temperatures the photons are not readily absorbed. As a result, the energy distribution and temperature are fairly uniform throughout the volume of hot gas. The fireball at this stage is consequently referred to as the “isothermal sphere. “ The name is something of a misnomer, since temperature gradients do exist, particularly near the advancing radiation front.
2.115 As the fireball cools, the transfer of energy by radiation and radiative growth become less rapid because of the decreasing mean free path of the photons. When the average temperature of the isothermal sphere has dropped to about 300,000°C, the expansion velocity will have decreased to a value comparable to the local acoustic (sound) velocity. At this point, a shock wave develops at the fireball front and the subsequent growth of the fireball is dominated by the shock and associated hydrodynamic expansion. The phenomenon of shock formation is sometimes called “hydrodynamic separation.” For a 20-kiloton burst it occurs at about a tenth of a millisecond after the explosion when the fireball radius is roughly 40 feet.
2.116 At very early times, beginning in less than a microsecond, an “inner” shock wave forms driven by the expanding bomb debris. This shock expands outward within the isothermal sphere at a velocity exceeding the local acoustic velocity. The inner shock overtakes and merges with the outer shock at the fireball front shortly after hydrodynamic separation. The relative importance of the debris shock wave depends on the ratio of the yield to the mass of the exploding device and on the altitude of the explosion (§ 2.136). The debris shock front is a strong source of ultraviolet radiation, and for weapons of small yield-to-mass ratio it may replace the X-ray fireball as the dominant energy source for the radiative growth.
2.117 As the (combined) shock front from a normal air burst moves ahead of the isothermal sphere it causes a tremendous compression of the ambient air and the temperature is thereby increased to an extent sufficient to render the air incandescent. The luminous shell thus formed constitutes the advancing visible fireball during this “hydrodynamic phase” of fireball growth. The fireball now consists of two concentric regions. The inner (hotter) region is the isothermal sphere of uniform temperature, and it is surrounded by a layer of luminous, shock-heated air at a somewhat lower, but still high, temperature. Because hot (over 8,000°C) air is effectively opaque to visible radiation, the isothermal sphere is not visible through the outer shocked air.
2.118 Some of the phenomena described above are represented schematically in Fig. 2.118; qualitative temperature profiles are shown at the left and pressure profiles at the right of a series of photographs of the fireball at various intervals after the detonation of a 20-kiloton weapon. In the first picture, at 0.1 millisecond, the temperature is shown to be uniform within the fireball and to drop abruptly at the exterior, so that the condition is that of the isothermal sphere. Subsequently, as the shock front begins to move ahead of the isothermal sphere, the temperature is no longer uniform, as indicated by the more gradual fall near the outside of the fireball. Eventually, two separate temperature regions form. The outer region absorbs the radiation from the isothermal sphere in the center and so the latter cannot be seen. The photographs, therefore, show only the exterior surface of the fireball.
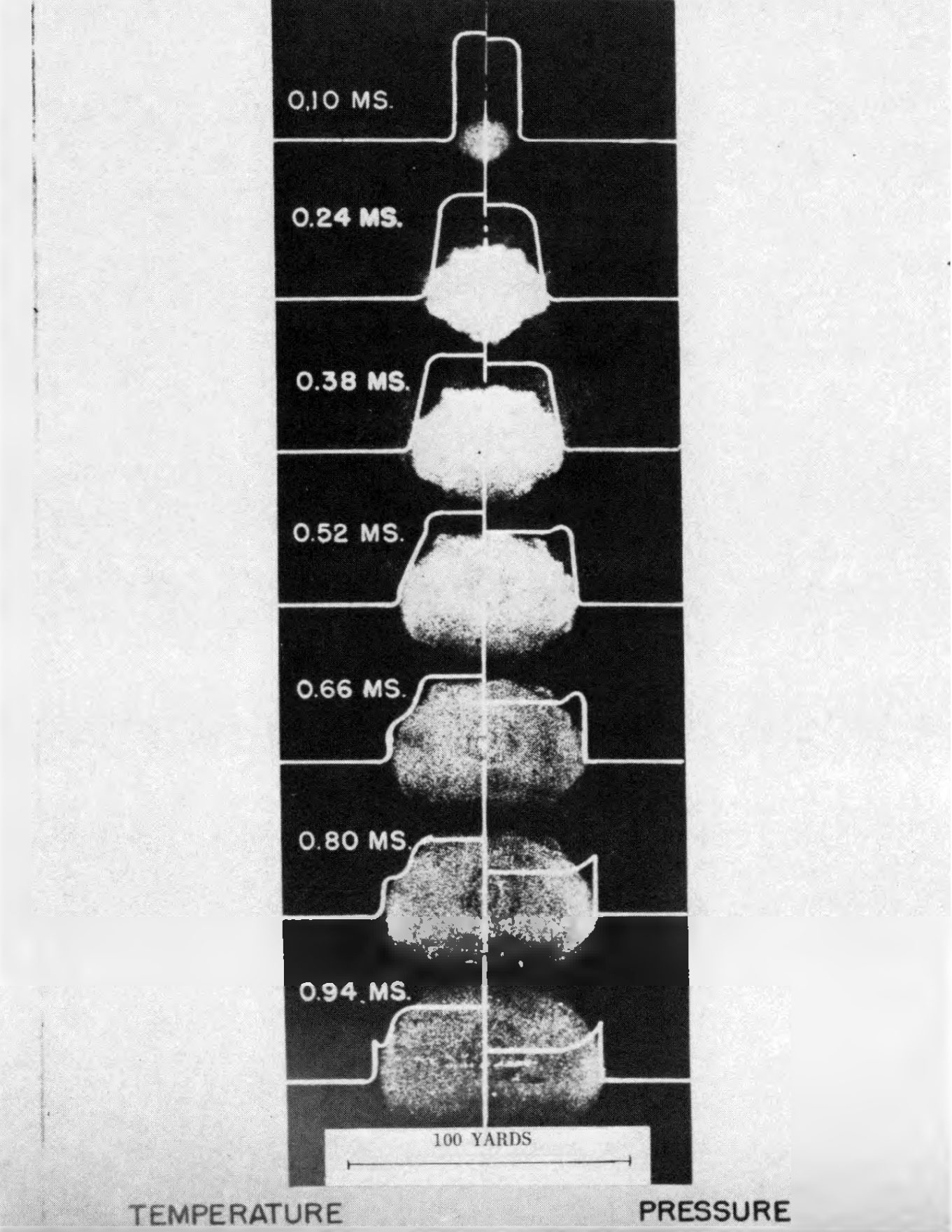
2.119 From the shapes of the curves at the right of Fig. 2.118 the nature of the pressure changes in the fireball can be understood. In the isothermal stage the pressure is uniform throughout and drops sharply at the outside, but after a short time, when the shock front has separated from the isothermal sphere, the pressure near the surface is greater than in the interior of the fireball. Within less than 1 millisecond the steep-fronted shock wave has traveled some distance ahead of the isothermal region. The rise of the pressure in the fireball to a peak, which is characteristic of a shock wave, followed by a sharp drop at the external surface, implies that the latter is identical with the shock front. It will be noted, incidentally, from the photographs, that the surface of the fireball, which has hitherto been somewhat uneven, has now become sharply defined.
2.120 For some time the fireball continues to grow in size at a rate determined by the propagation of the shock front in the surrounding air. During this period the temperature of the shocked air decreases steadily so that it becomes less opaque. Eventually, it is transparent enough to permit the much hotter and still incandescent interior of the fireball, i.e. , the isothermal sphere, to be seen through the faintly visible shock front (see Fig. 2.32). The onset of this condition at about 15 milliseconds (0.015 second) after the detonation of a 20-kiloton weapon, for example, is referred to as the “breakaway.”
2.121 Following the breakaway, the visible fireball continues to increase in size at a slower rate than before, the maximum dimensions being attained after about a second or so. The manner in which the radius increases with time, in the period from roughly 0.1 millisecond to 1 second after the detonation of a 20-kiloton nuclear weapon, is shown in Figure 2. 121. Attention should be called to the fact that both scales are logarithmic, so that the lower portion of the curve (at the left) does not represent a constant rate of growth, but rather one that falls off with time. Nevertheless, the marked decrease in the rate at which the fireball grows after breakaway is apparent from the subsequent flattening of the curve.
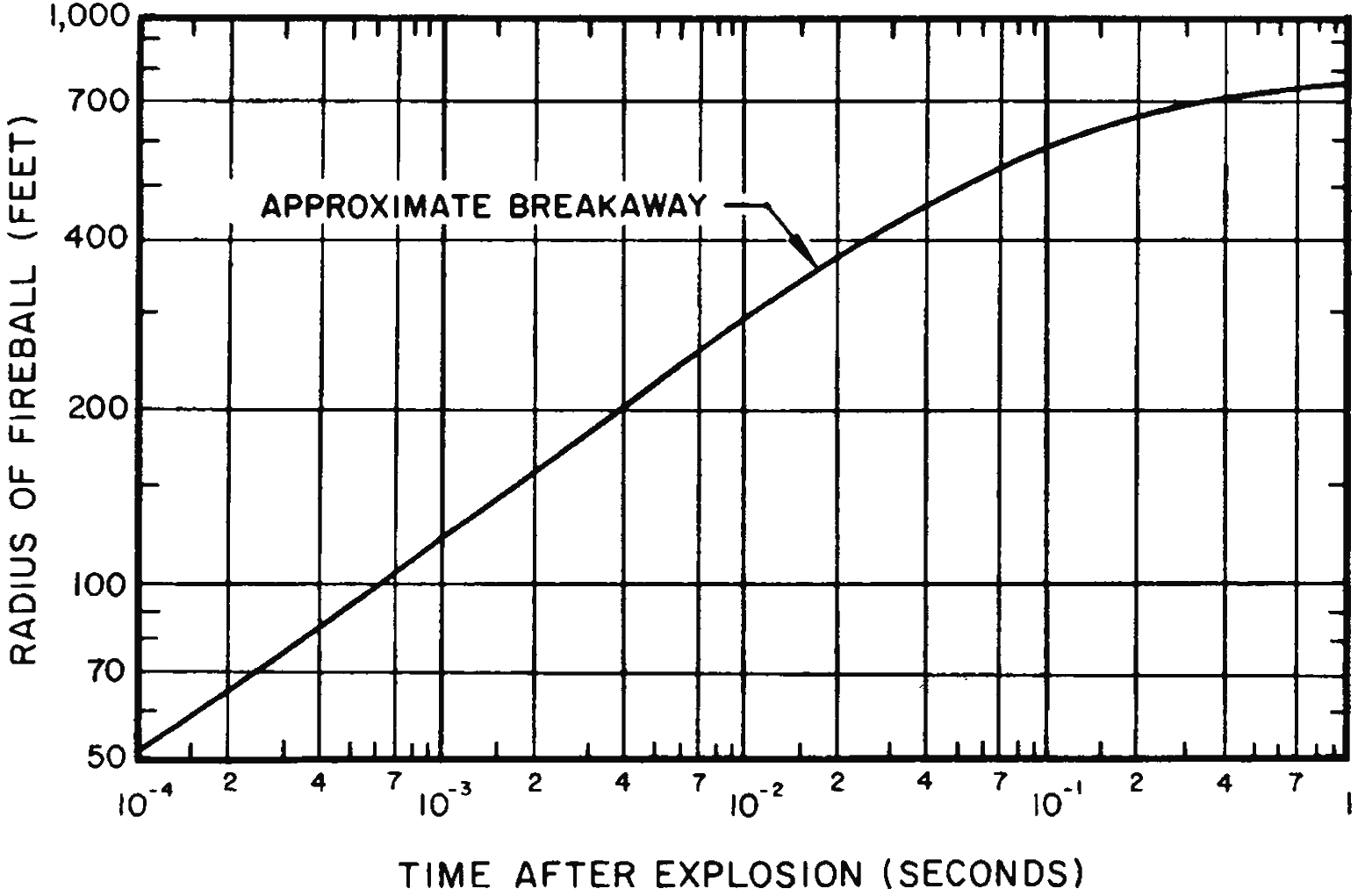
TEMPERATURE OF THE FIREBALL
2.122 As indicated earlier, the interior temperature of the fireball decreases steadily, but the apparent surface temperature, which influences the emission of thermal radiation, decreases to a minimum and then increases to a maximum before the final steady decline. This behavior is related to the fact that at high temperatures air both absorbs and emits thermal radiation very readily, but as the temperature falls below a few thousand degrees, the ability to absorb and radiate decreases.
2.123 From about the time the fireball temperature has fallen to 300,000°C, when the shock front begins to move ahead of the isothermal sphere, until close to the time of the first temperature minimum (§ 2.38), the expansion of the fireball is governed by the laws of hydrodynamics. It is then possible to calculate the temperature of the shocked air from the measured shock velocity, i.e., the rate of growth of the fireball. The variation of the temperature of the shock front with time, obtained in this manner, is shown by the full line from 10-4 to 10-2 second in Fig. 2.123, for a 20-kiloton explosion. But photographic and spectroscopic observations of the surface brightness of the advancing shock front, made from a distance, indicate the much lower temperatures represented by the broken curve in the figure. The reason for this discrepancy is that both the nuclear and thermal radiations emitted in the earliest stages of the detonation interact in depth with the gases of the atmosphere ahead of the shock front to produce ozone, nitrogen dioxide, nitrous acid, etc. These substances are strong absorbers of radiation coming from the fireball, so that the brightness observed some distance away corresponds to a temperature considerably lower than that of the shock front.
2.124 Provided the temperature of the air at the shock front is sufficiently high, the isothermal sphere is invisible (§ 2.117). The rate at which the shock front emits (and absorbs) radiation is determined by its temperature and radius. The temperature at this time is considerably lower than that of the isothermal sphere but the radius is larger. However, as the temperature of the shocked air approaches 3,000°C (5,400°F) it absorbs (and radiates) less readily. Thus the shock front becomes increasingly transparent to the radiation from the isothermal sphere and there is a gradual unmasking of the still hot isothermal sphere, representing breakaway (§ 2.120).
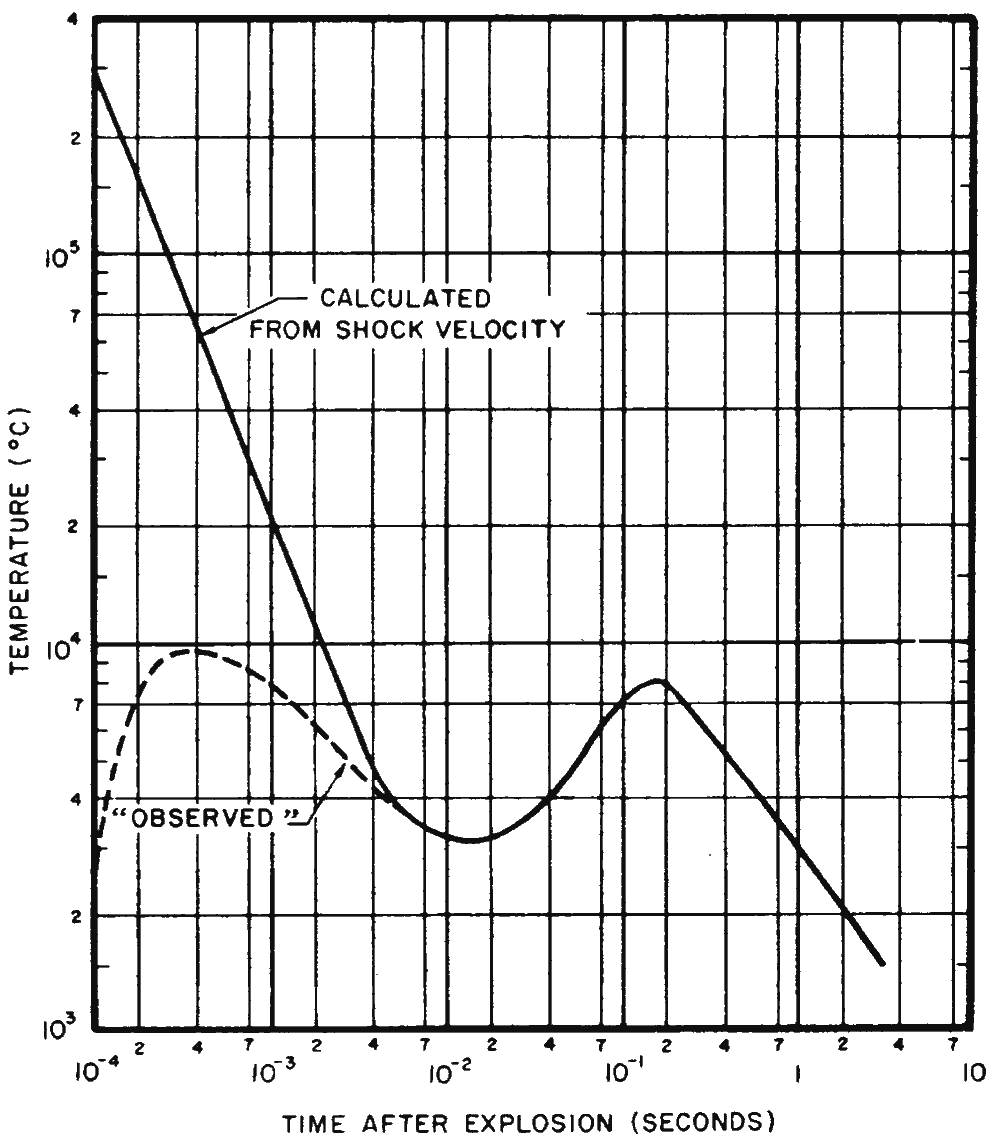
2.125 As a result of this unmasking of the isothermal sphere, the apparent surface temperature (or brightness) of the fireball increases (Fig. 2 .123), after passing through the temperature minimum of about 3,000°C attributed to the shock front. This minimum, representing the end of the first thermal pulse, occurs at about 11 milliseconds (0.011 second) after the explosion time for a 20-kiloton weapon. Subsequently, as the brightness continues to increase from the minimum, radiation from the fireball is emitted directly from the hot interior (or isothermal sphere), largely unimpeded by the cooled air in the shock wave ahead of it; energy is then radiated more rapidly than before. The apparent surface temperature increases to a maximum of about 7,700°C (14,000°F), and this is followed by a steady decrease over a period of seconds as the fireball cools by the emission of radiation and mixing with air. It is during the second pulse that the major part of the thermal radiation is emitted in an air burst (§ 2.38 et seq.). In such a burst, the rate of emission of radiation is greatest when the surface temperature is at the maximum.
2.126 The curves in Figs. 2.121 and 2.123 apply to a 20-kiloton nuclear burst, but similar results are obtained for explosions of other energy yields. The minimum temperature. of the radiating surface and the subsequent temperature maximum are essentially independent of the yield of the explosion. But the times at which these temperatures occur for an air burst increase approximately as the 0.4 power of the yield (Chapter VII). The time of breakaway is generally very soon after the thermal minimum is attained.
SIZE OF THE FIREBALL
2.127 The size of the fireball increases with the energy yield of the explosion. Because of the complex interaction of hydrodynamic and radiation factors, the radius of the fireball at the thermal minimum is not very different for air and surface bursts of the same yield. The relationship between the average radius and the yield is then given approximately by
where is the fireball radius in feet and is the explosion yield in kilotons TNT equivalent. The breakaway phenomenon, on the other hand, is determined almost entirely by hydrodynamic considerations, so that a distinction should be made between air and surface bursts. For an air burst the radius of the fireball is given by
For a contact surface burst, i.e., in which the exploding weapon is actually on the surface,8 blast wave energy is reflected back from the surface into the fireball (§ 3.34) and W in equation (2.127.1) should probably be replaced by 2 W, where W is the actual yield. Hence, for a contact surface burst,
For surface bursts in the transition range between air bursts and contact bursts, the radius of the fireball at breakaway is somewhere between the values given by equations (2.127.1) and (2.127.2). The size of the fireball is not well defined in its later stages, but as a rough approximation the maximum radius may be taken to be about twice that at the time of breakaway (cf. Fig. 2.121).
2.128 Related to the fireball size is the question of the height of burst at which early (or local) fallout ceases to be a serious problem. As a guide, it may be stated that this is very roughly related to the weapon yield by
where feet is the maximum value of the height of burst for which there will be appreciable local fallout. This expression is plotted in Fig. 2.128. For an explosion of 1,000 kilotons, i.e., 1 megaton yield, it can be found from Fig. 2.128 or equation (2.128.1) that significant local fallout is probable for heights of burst less than about 2,900 feet. It should be emphasized that the heights of burst estimated in this manner are approximations only, with probable errors of ±30 percent. Furthermore, it must not be assumed that if the burst height exceeds the value given by equation (2.128.1) there will definitely be no local fallout. The amount, if any, may be expected, however, to be small enough to be tolerable under emergency conditions.
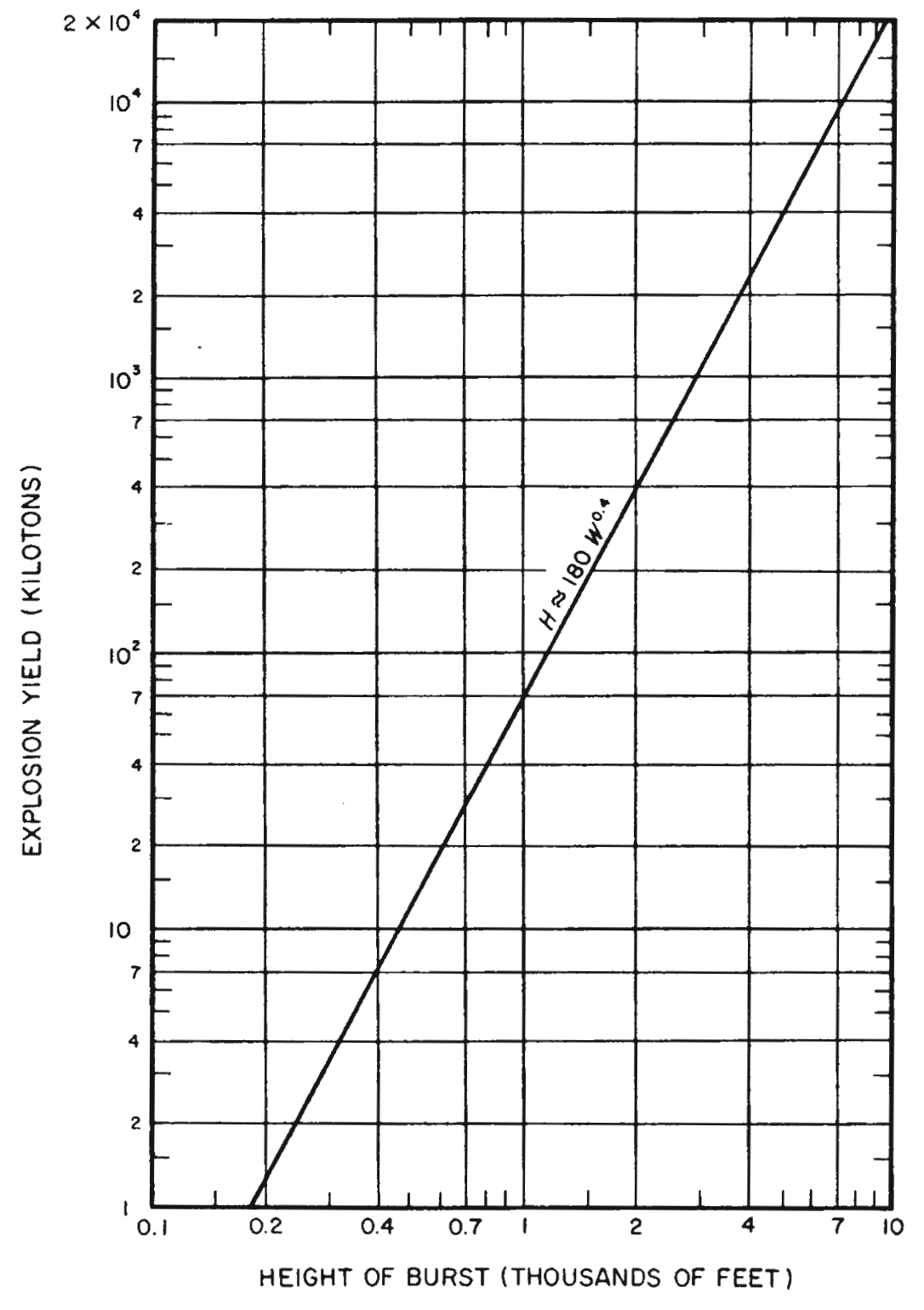
2.129 Other aspects of fireball size are determined by the conditions under which the fireball rises. If the fireball is small compared with an atmospheric scale height, which is about 4.3 miles at altitudes of interest (§ 10.123), the late fireball rise is caused by buoyant forces similar to those acting on a bubble rising in shallow water. This is called “buoyant” rise. The fireball is then essentially in pressure equilibrium with the surrounding air as it rises. If the initial fireball radius is comparable to or greater than a scale height, the atmospheric pressure on the bottom of the fireball is much larger than the pressure on the top. This causes a very rapid acceleration of the fireball, referred to as “ballistic” rise. The rise velocity becomes so great compared to the expansion rate that the fireball ascends almost like a solid projectile. “Overshoot” then occurs, in which a parcel of dense air is carried to high altitudes where the ambient air has a lower density. The dense “bubble” will subsequently expand, thereby decreasing its density, and will fall back until it is in a region of comparable density.
HIGH-ALTITUDE BURSTS
2.130 For nuclear detonations at heights up to about 100,000 feet (19 miles), the distribution of explosion energy between thermal radiation and blast varies only to a small extent with yield and detonation altitude (§ 1.24). But at burst altitudes above 100,000 feet, the distribution begins to change more noticeably with increasing height of burst (see Chapter VII). It is for this reason that the level of 100,000 feet has been chosen for distinguishing between air bursts and high-altitude bursts. There is, of course, no sharp change in behavior at this elevation, and so the definition of a high-altitude burst as being at a height above 100,000 feet hr somewhat arbitrary. There is a progressive decline in the blast energy with increasing height of burst above 100,000 feet, but the proportion of the explosion energy received as effective thermal radiation on the ground at first increases only slightly with altitude. Subsequently, as the burst altitude increases, the effective thermal radiation received on the ground decreases and becomes less than at an equal distance from an air burst of the same total yield (§ 7 .102).
2.131 For nuclear explosions at altitudes between 100,000 and about 270,000 feet (51 miles) the fireball phenomena are affected by the low density of the air. The probability of interaction of the primary thermal radiation, i.e., the thermal X rays, with atoms and molecules in the air is markedly decreased, so that the photons have long mean free paths and travel greater distances, on the average, before they are absorbed or degraded into heat and into radiations of longer wavelength (smaller photon energy). The volume of the atmosphere in which the energy of the radiation is deposited, over a period of a millisecond or so, may extend for several miles, the dimensions increasing with the burst altitude. The interaction of the air molecules with the prompt gamma rays, neutrons, and high-energy component of the X rays produces a strong flash of fluorescence radiation (§ 2.140), but there is less tendency for the X-ray veil to form than in an au burst (§ 2.111).
2.132 Because the primary thermal radiation energy in a high-altitude burst is deposited in a much larger volume of air, the energy per unit volume available for the development of the shock front is less than in an air burst. The outer shock wave (§ 2 .116) is slow to form and radiative expansion predominates in the growth of the fireball. The air at the shock front does not become hot enough to be opaque at times sufficiently early to mask the radiation front and the fireball radiates most of its energy very rapidly. There is no apparent temperature minimum as is the case for an air burst. Thus, with increasing height, a series of changes take place in the thermal pulse phenomena; the surface temperature minimum becomes less pronounced and eventually disappears, so that the thermal radiation is emitted in a single pulse of fairly short duration. In the absence of the obscuring opaque shock front, the fireball surface is visible throughout the period of radiative growth and the temperature is higher than for a low-altitude fireball. Both of these effects contribute to the increase in the thermal radiation emission.
2.133 A qualitative comparison of the rate of arrival of thermal radiation energy at a distance from the burst point as a function of time for a megaton range explosion at high altitude and in a sea-level atmosphere is shown in Fig. 2.133. In a low (or moderately low) air burst, the thermal radiation is emitted in two pulses, but in a high-altitude burst there is only a single pulse in which most of the radiation is emitted in a relatively short time. Furthermore, the thermal pulse from a high-altitude explosion is richer in ultraviolet radiation than is the main (second) pulse from an air burst. The reason is that formation of ozone, oxides of nitrogen, and nitrous acid (§ 2.123), which absorb strongly in this spectral region, is decreased.
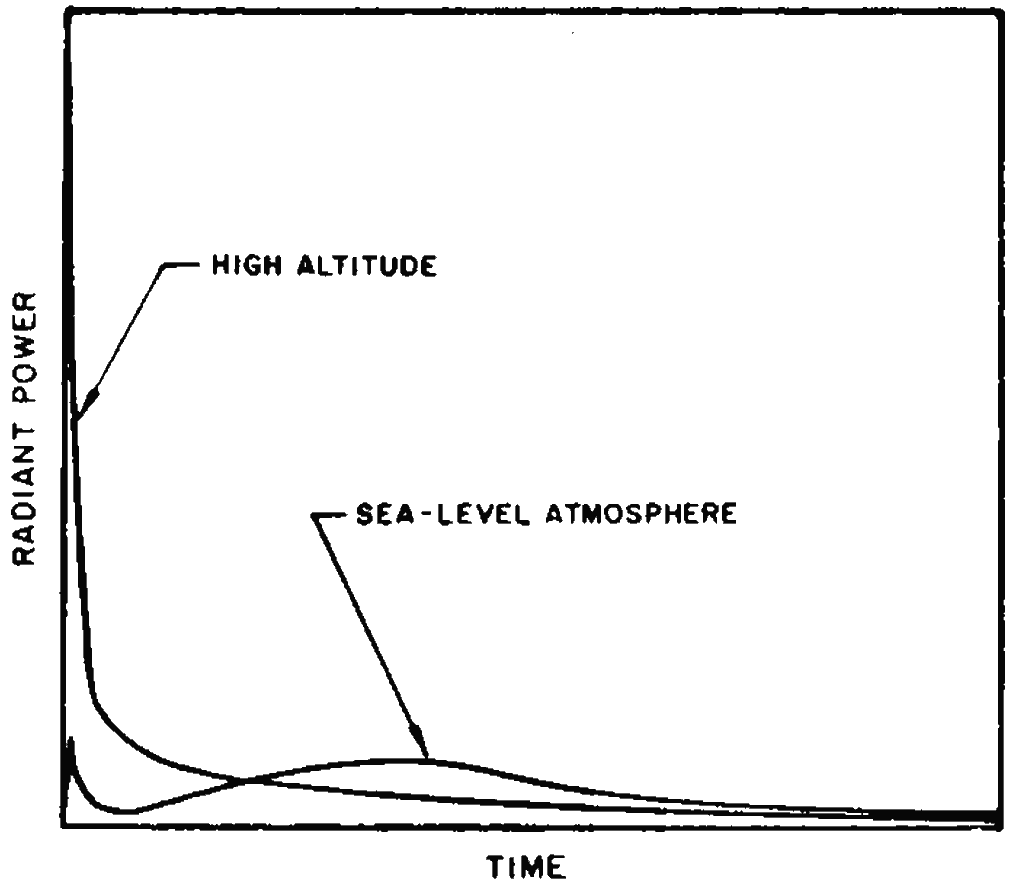
2.134 For burst altitudes above about 270,000 feet, there is virtually no absorption of the X rays emitted in upward directions. The downward directed X rays are mostly absorbed in a layer of air, called the “X-ray pancake,” which becomes incandescent as a result of energy deposition. The so-called pancake is more like the frustum of a cone, pointing upward, with a thickness of roughly 30,000 feet (or more) and a mean altitude of around 270,000 feet; the radius at this altitude is approximately equal to the height of burst minus 270,000 feet. The height and dimensions of the pancake are determined largely by the emission temperature for the primary X rays, which depends on the weapon yield and design, but the values given here are regarded as being reasonable averages. Because of the very large volume and mass of air in the X-ray pancake, the temperatures reached in the layer are much lower than those in the fireballs from bursts in the normal atmosphere. Various excited atoms and ions are formed and the radiations of lower energy (longer wavelength) re-emitted by these species represent the thermal radiation observed at a distance.
2.135 For heights of burst up to about 270,000 feet, the early fireball is approximately spherical, although at the higher altitudes it begins to elongate vertically. The weapon debris and the incandescent air heated by the X rays roughly coincide. Above 270,000 feet, however, the debris tends to be separate from the X-ray pancake. The debris can rise to great altitudes, depending on the explosion yield and the burst height; its behavior and ionization effects are described in detail in Chapter X. The incandescent (X-ray pancake) region, on the other hand, remains at an essentially constant altitude regardless of the height of burst. From this region the thermal radiation is emitted as a single pulse containing a substantially smaller proportion of the total explosion energy but of somewhat longer duration than for detonations below roughly 270,000 feet (see § 7.89 et seq.).
2.136 Although the energy density in the atmosphere as the result of a high-altitude burst is small compared with that from an air burst of the same yield, a shock wave is ultimately produced by the weapon debris (§ 2.116), at least for bursts up to about 400,000 feet (75 miles) altitude. For example, disturbance of the ionosphere in the vicinity of Hawaii after the TEAK shot (at 252,000 feet altitude) indicated that a shock wave was being propagated at that time at an average speed of about 4,200 feet per second. The formation of the large red, luminous sphere, several hundred miles in diameter, surrounding the fireball, has been attributed to the electronic excitation of oxygen atoms by the energy of the shock wave. Soon after excitation, the excess energy was emitted as visible radiation toward the red end of the spectrum (6,300 and 6,364 A).
2.137 For bursts above about 400,000 feet, the earth’s magnetic field plays an increasingly important role in controlling weapon debris motion, and it becomes the dominant factor for explosions above 200 miles or so (Chapter X). At these altitudes, the shock waves are probably magnetohydrodynamic (rather than purely hydrodynamic) in character. The amount of primary thermal radiation produced by these shock waves is quite small.
AIR FLUORESCENCE PHENOMENA
2.138 Various transient fluorescent effects, that is, the emission of visible and ultraviolet radiations for very short periods of time, accompany nuclear explosions in the atmosphere and at high altitudes. These effects arise from electronic excitation (and ionization) of atoms and molecules in the air resulting from interactions with high-energy X rays from the fireball, or with gamma rays, neutrons, beta particles, or other charged particles of sufficient energy. The excess energy of the excited atoms, molecules, and ions is then rapidly emitted as fluorescence radiation.
2.139 In a conventional air burst, i.e., at an altitude below about 100,000 feet, the first brief fluorescence that can be detected, within a microsecond or so of the explosion time, is called the “Teller light.” The excited particles are produced initially by the prompt (or instantaneous) gamma rays that accompany the fission process and in the later stages by the interaction of fast neutrons with nuclei in the air (§ 8.53).
2.140 For bursts above 100,000 feet, the gamma rays and neutrons tend to be absorbed, with an emission of fluorescence, in a region at an altitude of about 15 miles (80,000 feet), since at higher altitudes the mean free paths in the low-density air are too long for appreciable local absorption (§ 10.29). The fluorescence is emitted over a relatively long period of time because of time-of-flight delays resulting from the distances traveled by the photons and neutrons before they are absorbed. An appreciable fraction of the high-energy X rays escaping from the explosion region are deposited outside the fireball and also produce fluorescence. The relative importance of the X-ray fluorescence increases with the altitude of the burst point.
2.141 High-energy beta particles associated with bursts at sufficiently high altitudes can also cause air fluorescence. For explosions above about 40 miles, the beta particles emitted by the weapon residues in the downward direction are absorbed in the air roughly at this altitude, their outward spread being restricted by the geomagnetic field lines (§ 10.63 et seq.). A region of air fluorescence, called a “beta patch,” may then be formed. If the burst is at a sufficiently high altitude, the weapon debris ions can themselves produce fluorescence. A fraction of these ions can be channeled by the geomagnetic field to an altitude of about 70 miles where they are stopped by the atmosphere (§ 10.29) and cause the air to fluoresce. Under suitable conditions, as will be explained below, fluorescence due to beta particles and debris ions can also appear in the atmosphere in the opposite hemisphere of earth to the one in which the nuclear explosion occurred.
AURORAL PHENOMENA
2.142 The auroral phenomena associated with high-altitude explosions (§ 2.62) are caused by the beta particles emitted by the radioactive weapon residues and, to a varying extent, by the debris ions. Interaction of these charged particles with the atmosphere produces excited molecules, atoms, and ions which emit their excess energy in the form of visible radiations characteristic of natural auroras. In this respect, there is a resemblance to the production of the air fluorescence described above. However, auroras are produced by charged particles of lower energy and they persist for a much longer time, namely, several minutes compared with fractions of a second for air fluorescence. Furthermore, the radiations have somewhat different wavelength characteristics since they are emitted, as a general rule, by a different distribution of excited species.
2.143 The geomagnetic field exerts forces on charged particles, i.e., beta particles (electrons) and debris ions, so that these particles are constrained to travel in helical (spiral) paths along the field lines. Since the earth behaves like a magnetic dipole, and has north and south poles, the field lines reach the earth at two points, called “conjugate points,” one north of the magnetic equator and the other south of it. Hence, the charged particles spiraling about the geomagnetic field lines will enter the atmosphere in corresponding conjugate regions. It is in these regions that the auroras may be expected to form (Fig. 2.143).
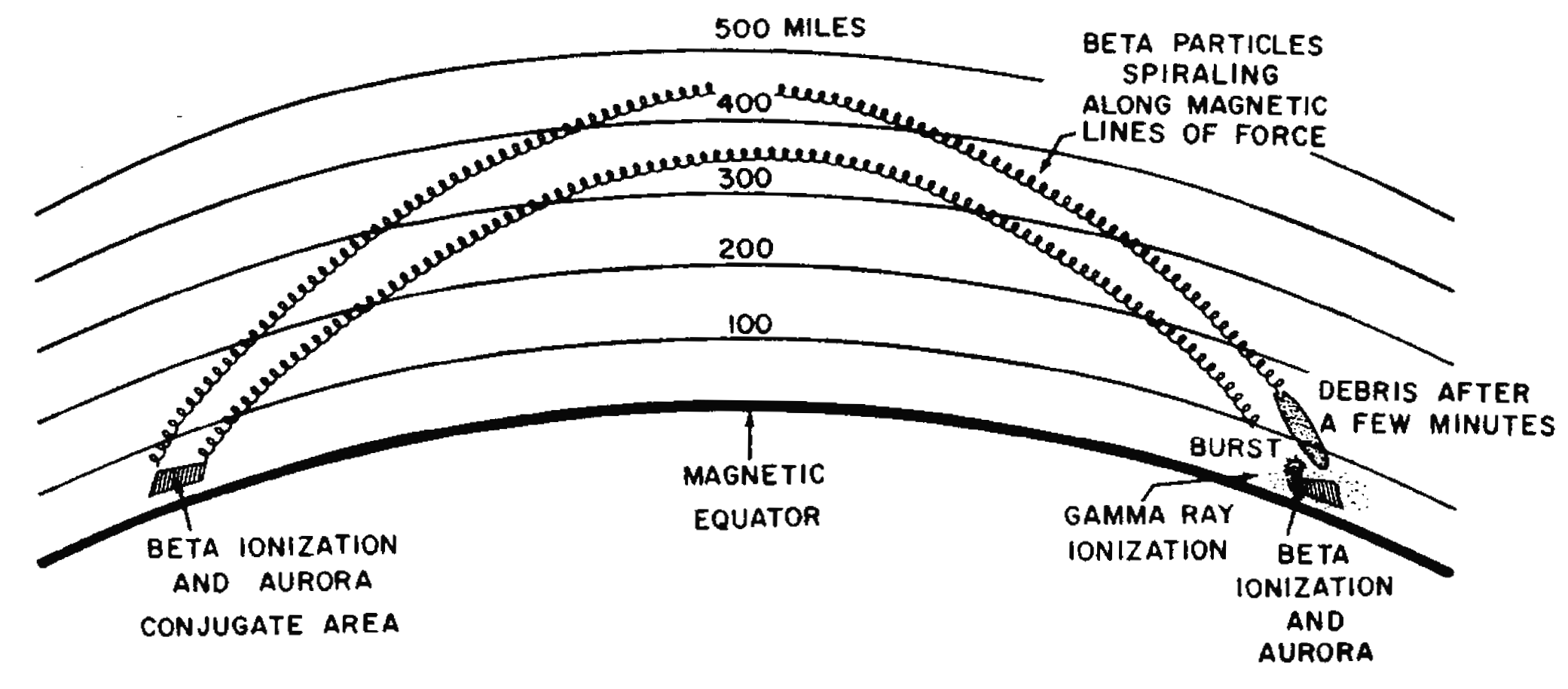
2.144 For the high-altitude tests conducted in 1958 and 1962 in the vicinity of Johnston Island (§ 2.52), the charged particles entered the atmosphere in the northern hemisphere between Johnston Island and the main Hawaiian Islands, whereas the conjugate region in the southern hemisphere region was in the vicinity of the Samoan, Fiji, and Tonga Islands. It is in these areas that auroras were actually observed, in addition to those in the areas of the nuclear explosions.
2.145 Because the beta particles have high velocities, the beta auroras in the remote (southern) hemisphere appeared within a fraction of a second of those in the hemisphere where the bursts had occurred. The debris ions, however, travel more slowly and so the debris aurora in the remote hemisphere, if it is formed, appears at a somewhat later time. The beta auroras are generally most intense at an altitude of 30 to 60 miles, whereas the intensity of the debris auroras is greatest in the 60 to 125 miles range. Remote conjugate beta auroras can occur if the detonation is above 25 miles, whereas debris auroras appear only if the detonation altitude is in excess of some 200 miles.
THE ARGUS EFFECT
2.146 For bursts at sufficiently high altitudes, the debris ions, moving along the earth’s magnetic field lines, are mostly brought to rest at altitudes of about 70 miles near the conjugate points. There they continue to decay and so act as a stationary source of beta particles which spiral about the geomagnetic lines of force. When the particles enter a region where the strength of the earth’s magnetic field increases significantly, as it does in the vicinity of the conjugate points, some of the beta particles are turned back (or reflected). Consequently, they may travel back and forth, from one conjugate region to the other, a number of times before they are eventually captured in the atmosphere. (More will be said in Chapter X about the interactions of the geomagnetic field with the charged particles and radiations produced by a nuclear explosion.)
2.147 In addition to the motion of the charged particles along the field lines, there is a tendency for them to move across the lines wherever the magnetic field strength is not uniform. This results in an eastward (longitudinal) drift around the earth superimposed on the back-and-forth spiral motion between regions near the conjugate points. Within a few hours after a high altitude nuclear detonation, the beta particles form a shell completely around the earth. In the ARGUS experiment (§ 2.53), in which the bursts occurred at altitudes of 125 to 300 miles, well defined shells of about 60 miles thickness, with measurable electron densities, were established and remained for several days. This has become known as the “ARGUS effect.” Similar phenomena were observed after the STARFISH PRIME (§ 2.52) and other high-altitude nuclear explosions.
EFFECT ON THE OZONE LAYER
2.148 Ozone (O3) is formed in the upper atmosphere, mainly in the stratosphere (see Fig. 9. 126) in the altitude range of approximately 50,000 to l00,000 feet (roughly 10 to 20 miles), by the action of solar radiation on molecular oxygen (O). The accumulation of ozone is limited by its decomposition, partly by the absorption of solar ultraviolet radiation in the wavelength range from about 2,100 to 3,000 A and partly by chemical reaction with traces of nitrogen oxides (and other chemical species) present in the atmosphere. The chemical decomposition occurs by way of a complex series of chain reactions whereby small quantities of nitrogen oxides can cause considerable breakdown of the ozone. The equilibrium (or steady-state) concentration of ozone at any time represents a balance between the rates of formation and decomposition; hence, it is significantly dependent on the amount of nitrogen oxides present. Solar radiation is, of course, another determining factor; the normal concentration of ozone varies, consequently, with the latitude, season of the year, time of day, the stage in the solar (sunspot) cycle, and perhaps with other factors not yet defined.
2.149 Although the equilibrium amount in the atmosphere is small, rarely exceeding 10 parts by weight per million parts of air, ozone has an important bearing on life on earth. If it were not for the absorption of much of the solar ultraviolet radiation by the ozone, life as currently known could not exist except possibly in the ocean. A significant reduction in the ozone concentration, e.g., as a result of an increase in the amount of nitrogen oxides, would be expected to cause an increased incidence of skin cancer and to have adverse effects on plant and animal life.
2.150 As seen in §§ 2.08 and 2.123, nuclear explosions are accompanied by the formation of oxides of nitrogen. An air burst, for example, is estimated to produce about 1032 molecules of nitrogen oxides per megaton TNT equivalent. For nuclear explosions of intermediate and moderately high yield in the air or near the surface, the cloud reaches into the altitude range of 50,000 to 100,000 feet (Fig. 2.16); hence, the nitrogen oxides from such explosions would be expected to enhance mechanisms which tend to decrease the ozone concentration. Routine monitoring of the atmosphere during and following periods of major nuclear testing have shown no significant change in the ozone concentration in the sense of marked, long-lasting perturbations. However, the large natural variations in the ozone layer and uncertainties in the measurements do not allow an unambiguous conclusion to be reached. Theoretical calculations indicate that extensive use of nuclear weapons in warfare could cause a substantial decrease in the atmospheric ozone concentration, accompanied by an increase in adverse biological effects due to ultraviolet radiation. The ozone layer should eventually recover, but this might take up to 25 years.
BIBLIOGRAPHY
- , “The Effect of Atmospheric Nuclear Explosions on Total Ozone,” Institute for Defense Analyses, January 1975, Paper P–1076, IDA Log. No. HQ 74–16726.
- * , et al., “Blast Wave,” University of California, Los Alamos Scientific Laboratory, March, 1958, LA–2000.
- , “Conjugate Auroral Measurements from the 1962 U.S. High Altitude Nuclear Test Series,” in “Aurora and Airglow,” B. M. McCormac, Ed., Reinhold Publishing Corp., 1967.
- , “Temperature in Atomic Explosions,” in “Temperature, Its Measurement and Control in Science and Industry,” Reinhold Publishing Corp., 1955, Vol. II, p. 395.
- , “Review of Nuclear Weapons Effects,” Ann Rev. Nuclear Science, 18, 153 (1968).
- , “The Argus Experiment,” J. Geophys. Res., 64, 869 (1959). FOLEY, N. M., and M. A. RUDERMAN, “Stratospheric Nitric Oxide Production from Past Nuclear Explosions and Its Relevance to Projected SST Pollution,” Institute for Defense Analyses, August 1972, Paper P–894, IDA Log. No. HQ 72–14452.
- , “The Production of Nitrogen Oxides by Low Altitude Nuclear Explosions,” Institute for Defense Analyses, July 1974, Paper P–986, IDA Log. No. HQ 73–15738.
- , “Public Safety and Underground Nuclear Detonations,” U.S. Atomic Energy Commission, June 1971, TID–25708.
- , Ed., “Collected Papers on the Artificial Radiation Belt from the July 9, 1962 Nuclear Detonation,” J. Geophys. Res., 68, 605 et seq. (1963).
- * , “United States High-Altitude Test Experiences,” University of California, Los Alamos Scientific Laboratory, October 1976, LA405.
- * , “Phenomenology of Contained Nuclear Explosions,” University of California, Lawrence Radiation Laboratory, Livermore, December 1958, UCRL 5124 Rev. 1.
- * , et al., “Underground Nuclear Detonations,” University of California, Lawrence Radiation Laboratory, Livermore, July 1959, UCRL 5626.
- , “On Artificial Geomagnetic and Ionospheric Storms Associated with High Altitude Explosions,” J. Geophys. Res., 64, 1149 (1959).
- , “EMP Impact on U.S. Defense,” Survive, 2, No. 6, 2 (1969).
- * “Proceedings of the Third Plowshare Symposium, Engineering with Nuclear Explosives,” April 1966, University of California, Lawrence Radiation Laboratory, Livermore, TID–695.
- ”Proceedings of the Symposium on Engineering with Nuclear Explosives,” Las Vegas, Nevada, January 1970, American Nuclear Society and U.S. Atomic Energy Commission, CONF–700101, Vols. 1 and 2.
- ”Proceedings for the Symposium on Public Health Aspects of Peaceful Uses of Nuclear Explosives,” Las Vegas, Nevada, April 1969, Southwestern Radiological Health Laboratory, SWRHL–82.
- ”Proceedings of the Special Session on Nuclear Excavation,” Nuclear Applications and Technology, 7, 188 et seq. (1969).
- , “Charts for the Parameters of Migrating Explosion Bubbles,” U.S. Naval Ordnance Laboratory, 1962, NOLTR 62–184.
- , “Photographs of the High Altitude Nuclear Explosion TEAK,” J. Geophys. Res., 65, 545 (1960).
- , “Blast Phenomena from Explosions and an Air-Water Interface,” U.S. Army Engineer Waterways Experiment Station, 1966, Report 1, Misc. Paper 1–814.
- , et al., “The Constructive Uses of Nuclear Explosives,” McGraw-Hill Book Company, 1968.
- , “Handbook of Explosion-Generated Water Waves, Vol. I—State of the Art,” Tetra Tech, Inc., Pasadena, California, October 1968, Report No. TC–130.
* These documents may be purchased from the National Technical Information Center, U.S. Department of Commerce, Springfield, Virginia, 22161.
ENDNOTES
- 1 A millisecond is a one-thousandth part of a second. [ref. § 2.04] X
- 2 The tropopause is the boundary between the troposphere and the relatively stable air of the stratosphere. It varies with season and latitude, ranging from 25,000 feet near the poles to about 55,000 feet in equatorial region (§ 9.128). [ref. § 2.13] X
- 3 These residues include radioactive species formed at the time of the explosion by neutron capture in various materials (§ 9.31). [ref. § 2.23] X
- 4 A micrometer (also called a micron) is a one-millionth part of a meter, i.e., 10-6 meter, or about 0.00004 (or ) inch. [ref. § 2.27] X
- 5 The term “ground zero” refers to the point on the earth’s surface immediately below (or above) the point of detonation. For a burst over (or under) water, the corresponding point is generally called “surface zero.” The term “surface zero” or “surface ground zero” is also commonly used for ground surface and underground explosions. In some publications, ground (or surface) zero is called the “hypocenter” of the explosion. [ref. § 2.34] X
- 6 The normal atmospheric pressure at sea level is 14.7 pounds per square inch. [ref. § 2.34] X
- 7 The remaining (more technical) sections of this chapter may be omitted without loss of continuity. [ref. Section 6] X
- 8 For most purposes, a contact surface burst may be defined as one for which the burst point is not more than 5 feet above or below the surface. [ref. § 2.127] X